Cardiopulmonary Resuscitation
Joseph P. Ornato
Mary Ann Peberdy
Overview
Closed-chest cardiopulmonary resuscitation (CPR), first described in 1960, circulates blood throughout the body by two different mechanisms: direct cardiac compression (the cardiac pump) and changes in intrathoracic pressure (the thoracic pump). Minimally interrupted chest compression along with a short “hands-off” interval can improve resuscitation outcome. Hyperventilation should be avoided during resuscitation.
The most common victim of sudden, unexpected cardiac arrest is a man between 50 and 75 years of age who has underlying coronary artery disease. The most common initial rhythm is pulseless ventricular tachycardia or ventricular fibrillation.
The most effective approach to resuscitation is to establish a strong chain of survival with early access to emergency care, early cardiopulmonary resuscitation, early defibrillation, and early advanced cardiac life support. Public access to defibrillation can strengthen the early-defibrillation link in the chain.
A variety of monitoring techniques can, and should, be used during clinical resuscitation.
There is little evidence that high-dose epinephrine is any better than standard 1-mg doses of epinephrine. Vasopressin, alone or alternating with epinephrine, is an alternative to epinephrine in the adult. Sodium bicarbonate is of very limited value in most cardiac arrest cases, unless hyperkalemia is present or the patient has taken an overdose of a tricyclic antidepressant or barbiturates. Intravenous amiodarone is the preferred antiarrhythmic agent for treating pulseless ventricular tachycardia or ventricular fibrillation.
Several promising new cardiopulmonary resuscitation techniques are undergoing development and clinical testing. In-hospital rapid response teams can decrease the number of cases that progress to cardiac arrest. Mild hypothermia induced after successful resuscitation appears to improve neurologically intact survival. Public access defibrillation has the potential to double survival from cardiac arrests that occur in public places.
Glossary
Automated external defibrillator (AED)
A defibrillator that automatically determines the cardiac rhythm and advises rescuers of the need to defibrillate using voice prompts. Fully automated devices charge and deliver the defibrillation energy with no input from the user. Semiautomated devices prompt the user to defibrillate when ventricular fibrillation is detected by the device.
Chain of survival
A coordinated system for treating victims of cardiac arrest that provides early access to emergency medical services, early cardiopulmonary resuscitation, early defibrillation, and early advanced cardiac life support.
High-dose epinephrine
Doses greater than 1.0 mg in adults (typically 3 to 15 mg).
Medical emergency team (MET)
A system that identifies and treats early clinical deterioration in hospitalized patients before the development of cardiac arrest.
Public access defibrillation
The use of automated external defibrillators by laypersons.
Pulseless electrical activity (PEA)
During cardiac arrest, an organized cardiac rhythm that does not generate a pulse. Previously known as electromechanical dissociation (EMD).
Historical perspective
Numerous and often barbaric methods for restoring life to the newly deceased have been attempted for centuries (1). It was not until about 50 years ago that the era of modern-day cardiopulmonary resuscitation (CPR) was born. The field of resuscitation is an integrated science combining chest compression, ventilation, defibrillation, and pharmacotherapeutics and has widespread use in both the prehospital and in-hospital arenas. Although it is difficult for us to imagine these elements independently, that is precisely how they began.
Artificial Ventilation
Nearly 200 years of effort and experimentation aimed at finding an effective method of artificial respiration culminated in the work of Archer Gordon in 1949. To compare various methods of artificial respiration, Gordon spent 1 year, with no salary and no funding, in Cook County Hospital, Chicago, where he was on call 24 hours/day. His experimental procedure consisted in inserting a tube into the trachea of a fresh corpse and performing multiple posturing techniques such as lifting the arms and pressing on the back or chest, lifting the hips, and rocking the deceased patient. He then observed the effects of these procedures via a respirometer connected to the tracheal tube. His results were first published in the Journal of the American Medical Association in 1950 (2).
In 1951, Gordon performed similar studies on medical students anesthetized with curare. Later that year he published a wealth of data (3) supporting the notion that these techniques were able to provide some degree of manual respiration. There were two limitations to Gordon’s relentless efforts. The first was that he only alluded to the idea of mouth-to-mouth ventilation and did not evaluate this technique, and the second was that all of his data were collected in intubated patients in whom the airway was fully protected.
Simultaneous with these efforts, James Elam discovered that by merely using expired air one could oxygenate humans adequately. His discovery was born of a need to ventilate patients suffering from acute paralysis during a polio epidemic in 1946. His repeated experience in performing mouth-to-nose breathing over a period of several years convinced him of its value. To document his beliefs scientifically, in 1954 Elam studied intubated, anesthetized, nonbreathing postoperative patients. He merely blew into their tracheal tubes with his own exhaled air and documented that by doing this he could maintain normal arterial oxygen saturation.
It was not until 1956 that fate brought Elam and his data together with Peter Safar. Lively discussion enticed Safar to begin experiments on mouth-to-mouth artificial respiration. By 1957, Safar had proven that the mouth-to-mouth technique was clearly superior to the manual ventilation techniques that were currently employed and that it could be performed quite easily by laypersons (4). He was also able to document that tilting a person’s head backward usually maintained the airway open, thus supporting previous data from patients with protected airways. By 1958, the work of Gordon, Elam, and Safar persuaded the American Medical Association and the U.S. Military to adopt the mouth-to-mouth technique as the official means of providing artificial respiration.
Defibrillation
Use of electrotherapy was a common practice for a variety of ailments ranging from paresis to death throughout much of the eighteenth and nineteenth centuries. Unfortunately, little was known about electrical forces or the ailments it purportedly treated, and there was no benefit to survival from its use. In fact, it took several decades to link electricity to the appropriate treatment for many causes of sudden death. Until the development of the electrocardiograph, ventricular fibrillation was a diagnosis that could only be made by observing the heart. It was first described in 1850 by Karl Ludwig in the dog lab (5). Ludwig noted that he could induce ventricular fibrillation by applying galvanic currents directly to a dog’s heart. Although this was likely the first link between electricity and sudden death, the connection was ignored for decades. In 1889, John McWilliam refuted the notion that sudden cardiac death was caused by ventricular standstill by describing a fibrillating heart in the dog lab. He documented, as others had before him, that electricity could induce this event in the canine model (6). Unfortunately, he never attempted to use electricity to terminate the events. Although he postulated that a similar scenario might occur in humans, he did not pursue this. It was not until a decade later that two French physiologists, Frederic Battelli and Jean Louis Prevost, reported that, although a weak current passed through the heart could cause fibrillation, a stronger current was capable of terminating it (7). This singularly important discovery remained dormant for the next several decades.
In the late 1920s the Electric Company and Edison Power Company reconsidered the idea of coupling electricity with defibrillation. They commissioned William Kouwenhoven, Donald Hooker, and Orthello Langworthy at the Johns Hopkins University to research electrical effects on the heart. This group quickly documented that weak shocks could induce ventricular fibrillation and stronger shocks could eradicate it. They were the first to discover that defibrillation could be successfully performed without opening the chest (8). The group also coined the term “countershock,” which was used synonymously with defibrillation. An initial shock placed the heart into ventricular fibrillation and a subsequent countershock could terminate it. None of these researchers pursued the possibility of using this countershock in humans to terminate ventricular fibrillation.
Two decades passed until Claude Beck successfully defibrillated a patient. Based on the work of Kouwenhoven, Hooker, and Langworthy, Beck constructed an alternating current (AC) internal defibrillator. Although he was successful in resuscitating a pediatric patient who had developed ventricular fibrillation during cardiac surgery, it took another 8 years for him to successfully use his device on another patient.
In 1955, Paul Zoll developed an external alternating current defibrillator. On a case- by-case basis, he documented that external defibrillation could be performed safely and effectively (9).
Defibrillators utilizing alternating current were quite heavy and cumbersome. It was very difficult to transport this equipment to needy patients in an emergency situation. In 1960, Bernard Lown developed a direct current (DC) defibrillator that was battery operated and readily transportable. This piece of equipment was the first in a long line of modern direct current defibrillators.
Closed-Chest Compressions
The first documented case of closed-chest compression for the treatment of cardiac arrest in the United States was in 1904 (10). Without the aid of defibrillation or pacing to restart the heart the concept was not particularly useful, and it never gained extensive popularity. It was not until the late 1950s that the technique of closed-chest massage was rediscovered. William Kouwenhoven and Guy Knickerbocker were studying fibrillation in their animal laboratory at the Johns Hopkins University. While performing experiments to determine how long a canine’s heart could be left fibrillating and still be successfully cardioverted, Knickerbocker noted that when he placed the defibrillator paddles snugly against the closed chest of the dog there was a slight increase in arterial pressure. The investigators noted that by applying the paddles in a repetitive motion they could prolong the time that the heart could stay in fibrillation and be defibrillated successfully. James Jude joined the group, and the team began performing multiple studies using the hands to compress the chest. Through a series of trial and error experiments, it was determined that applying a downward pressure of 1 1/2 to 2 inches on the lower sternum at a rate of 60 to 80 per minute was the optimal way to perform this maneuver. The investigators published data derived from patients suffering from in-hospital cardiac arrest in 1960. Fourteen of 20 patients survived to discharge from the hospital (11). It was finally understood that closed-chest compressions could “buy time” for the patient with a fibrillating heart until an external defibrillator arrived.
Modern-Day Cardiopulmonary Resuscitation
It was not until Safar, Kouwenhoven, and Knickerbocker presented their scientific data at the Maryland Medical Society meeting on September 16, 1960, that artificial ventilation was combined with artificial circulation to create CPR. The trio lectured around the world to promote the concept of CPR. Gordon produced a training film in 1962 and coined the mnemonic ABC for airway, breathing, circulation. The popularity of this technique, which could be performed by anyone and anywhere, spread quickly.
The American Heart Association officially endorsed CPR in 1963 and developed a CPR Committee. In 1966 the National Research Council of the National Academy of Sciences brought together representatives from more than 30 organizations, including the American Red Cross, and established official recommendations regarding standardized training and performance of CPR (12). Since then, the American Heart Association has hosted special conferences in 1973, 1979, 1985, 1992, 2000, and 2005 to establish consensus recommendations for resuscitation. Each of these conferences was followed by publication of educational documents that have been used worldwide to teach the science of resuscitation.
Pathophysiology
The Mechanism of Blood Flow During Cardiopulmonary Resuscitation
For 15 years after the discovery of closed-chest compression, it was generally believed that blood flow during CPR is caused by direct compression of the heart between the sternum and the spine (“cardiac pump” theory). In the 1970s, the “cardiac pump” theory was challenged by investigators who observed that increased intrathoracic pressure alone (without precordial compression) can generate blood flow. Criley et al. (13) reported that increases in intrathoracic pressure by repeated coughing could maintain adequate systemic blood pressure and flow to maintain consciousness without precordial compression in a patient who developed ventricular fibrillation during cardiac catheterization. This observation was confirmed by Niemann et al. (14), lending further support to the notion that pressurization of the thoracic cavity could generate blood flow and pressure by forcing blood out of both the heart and thoracic vessels. Sudden increase in the intrathoracic pressure causes air trapping in the alveoli and small bronchioles during chest compression, creating a pressure gradient between the intrathoracic and extrathoracic cavities (15). According to this “thoracic pump” theory, the heart functions as a passive conduit (16). Pressurization of the thorax collapses veins at the thoracic inlet, preventing venous backflow (17). Forward flow occurs because the more muscular arteries remain open, particularly if epinephrine is administered exogenously.
The relative contributions of the cardiac and thoracic pump mechanisms have been debated (16,18,19,20). Transesophageal echocardiography studies during cardiopulmonary resuscitation demonstrate that both mechanisms are operative in humans (19,21,22,23,24). Sternal compression virtually always squeezes blood out of the right ventricle (cardiac pump), whereas the amount of left ventricular compression is highly variable among patients (22). Physiologic studies in experimental models (25) and humans (26) suggest a strong role for the thoracic pump. In addition, active decompression of the chest by application of negative pressure or suction to the sternum can further enhance cardiac output by improving venous inflow (27,28,29,30,31,32). Finally, interposing an abdominal compression between each pair of chest compressions has also been tried in an attempt to mimic the physiologic effects of intraaortic balloon counterpulsation (27,33,34,35,36,37,38,39,40,41,42,43,44,45,46,47,48,49,50,51,52,53,54,55).
Three Phases of Resuscitation
Weisfeldt and Becker postulated that there are three physiologic phases of resuscitation: electrical, circulatory, and metabolic (56). When cardiac arrest occurs due to ventricular fibrillation, myocardial cells are rich in adenosine triphosphate (ATP) for the first 3 to 4 minutes (electrical phase). During the first few minutes, prompt defibrillation may be all that is required to restore the circulation. However, fibrillating myocardial cells consume ATP at a nearly normal rate (57). After several minutes, myocardial ATP stores are reduced sufficiently so that a defibrillation shock will ablate the ventricular fibrillation but will usually result in either asystole or pulseless electrical activity (PEA). During this “circulatory phase,” a brief (90 seconds to 3 minutes) period of effective CPR before defibrillation will boost myocardial ATP stores and increase the likelihood that a perfusing rhythm will result (58). This “CPR-first” strategy is now being used increasingly for patients whose cardiac arrest was not witnessed by trained health-care providers (59,60). The standard “shock-first” strategy is still advisable when a patient is witnessed to go into ventricular fibrillation and defibrillation can be accomplished within the first 3 to 4 minutes. If the patient remains in cardiac arrest from more than approximately 8 minutes, increasing ischemic cellular injury occurs that currently cannot be corrected with chest defibrillation and conventional CPR alone. This period is termed the “metabolic phase” of resuscitation, indicating that cellular-protective measures will likely be needed for successful recovery of vital organ function.
Minimal interruption of chest compression during resuscitation is important to maximize tissue oxygen delivery and, hence, myocardial ATP reserves. Unfortunately, chest
compressions are not maintained for as much as 40% to 50% of the time during a typical resuscitation, particularly if automated external defibrillators (AEDs) are used (61). The odds of success increase when continuous, or nearly continuous, chest compressions are performed during resuscitation (62). Shortening the time interval from the last chest compression until a defibrillation shock is administered (“hands-off interval”) can also increase the likelihood that a defibrillation shock will result in return of spontaneous circulation (ROSC) (63,64). It is important to ensure that complete chest wall decompression occurs during the upstroke on the sternum to improve venous filling (65). Finally, recent evidence suggests that hyperventilation may be harmful during resuscitation because it increases intrathoracic pressure, causing a marked decrease in venous filling. This paradoxically reduces cardiac output and tissue oxygen delivery (66,67).
compressions are not maintained for as much as 40% to 50% of the time during a typical resuscitation, particularly if automated external defibrillators (AEDs) are used (61). The odds of success increase when continuous, or nearly continuous, chest compressions are performed during resuscitation (62). Shortening the time interval from the last chest compression until a defibrillation shock is administered (“hands-off interval”) can also increase the likelihood that a defibrillation shock will result in return of spontaneous circulation (ROSC) (63,64). It is important to ensure that complete chest wall decompression occurs during the upstroke on the sternum to improve venous filling (65). Finally, recent evidence suggests that hyperventilation may be harmful during resuscitation because it increases intrathoracic pressure, causing a marked decrease in venous filling. This paradoxically reduces cardiac output and tissue oxygen delivery (66,67).
Clinical Profile of Sudden, Unexpected Cardiac Arrest
Most episodes of sudden, unexpected cardiac arrest in adults occur in the home or workplace (68,69,70,71). The most common victim is a man between 50 and 75 years of age (68,70). The majority of these individuals have significant underlying structural heart disease such as coronary artery atherosclerosis, hypertension, left ventricular hypertrophy, and/or congestive heart failure (69,72,73,74,75).
Most cardiac arrests occur without an obvious immediate precipitant, although many families of cardiac arrest victims report that the patient experienced a variety of nonspecific symptoms such as chest pain, dyspnea, fatigue, or malaise in the days preceding the event (69,72). On rare occasions, a dramatic emotional event can trigger cardiac arrest (76,77,78,79,80).
There is a definite circadian pattern to the onset of sudden, unexpected, out-of-hospital cardiac arrest. The incidence of cardiac arrest is lowest during sleep and begins to rise rapidly soon after awakening (81,82,83). Heart rate variability studies show that, at such times, vagal activity is relatively low and sympathetic activity is high, especially in patients with reduced left ventricular function (84).
In greater than 80% of patients who develop out-of-hospital, primary cardiac arrest during ambulatory electrocardiographic monitoring the initiating event is a ventricular tachyarrhythmia (ventricular tachycardia) degenerating rapidly to ventricular fibrillation in 62% of cases, torsades de pointes in 13%, and “primary” ventricular fibrillation in 8% (85). Fewer than 70% of patients are in ventricular tachycardia or ventricular fibrillation by the time rescue personnel arrive on the scene (typically 5 to 10 minutes after the onset of collapse in most efficient emergency medical services [EMS] systems) (86). Most of the remaining patients (31%) are in a pulseless, bradycardic rhythm or asystole.
The outcome of field resuscitation is strongly influenced by the patient’s initial cardiac rhythm. In one series of 352 consecutive out-of-hospital cardiac arrest patients, 67% of the patients with ventricular tachycardia and 23% of those who were initially in ventricular fibrillation survived to hospital discharge (87). None of the patients who presented with an initial bradyarrhythmia survived to hospital discharge. Similar observations have been made by others (88). One plausible hypothesis is that pulseless bradycardia or asystole may be a marker for a prolonged downtime interval or a more severe underlying disease process (89). Because ventricular tachyarrhythmias represent the most common potentially treatable mechanism of sudden cardiac arrest in adults, the best in-hospital and out-of-hospital resuscitation programs have been designed to deliver rapid defibrillation to as many patients as possible.
Principles of Management
The “Chain of Survival” Approach to Treatment
Survival from pulseless ventricular tachycardia or ventricular fibrillation is inversely related to the time interval between its onset and termination. Each minute that a patient remains in ventricular fibrillation, the odds of survival decrease by 7% to 10% (90). Survival is highest when CPR is started within the first 4 minutes of arrest and advanced cardiac life support (ACLS), including defibrillation and drug therapy, is started within the first 8 minutes (91). The American Heart Association’s Emergency Cardiovascular Care Committee and its Advanced Cardiovascular Life Support Subcommittee began to widely publicize the “chain of survival” concept in 1991. This phrase represents a sequence of events that should occur in most cardiac arrest cases to maximize the odds of successful resuscitation (90). The steps include early recognition of the problem and activation of the EMS system by a bystander, early CPR, rapid provision of defibrillation for patients who need it, and advanced cardiac life support (e.g., intubation, administration of medications). Schematically, this sequence can be depicted as a “chain of survival” (Fig. 69.1).
Early Access
The victim is rarely in a position to activate the EMS system before his or her collapse because most out-of-hospital cardiac arrests occur suddenly and without immediate premonitory symptoms. The same problem exists for in-hospital cardiac arrests that occur in unmonitored patients. Bystanders who witness the event can significantly improve the victim’s chance to survive by alerting the emergency response system to the presence of the problem. All too often, an untrained bystander only further delays treatment of the out-of-hospital cardiac arrest victim by attempting to inform relatives, call the neighbors, or contact the patient’s personal physician instead of calling the local community emergency telephone number (in most places it is 911) (92,93).
Before EMS rescuers can aid the victim, the bystander must recognize that there is a problem, locate a telephone, make a correct call, and give accurate and precise information to the dispatcher. Once the alarm has been sounded, rescuers must travel to the scene, arrive at the patient’s side, and perform an initial, cursory assessment.
Public education can improve bystander behavior significantly when a cardiac emergency occurs in the community. Citizens can be trained to summon help quickly and to initiate lifesaving CPR. The American Heart Association and the American Red Cross have trained millions of citizens to recognize cardiac emergencies, call for help, and perform CPR.
Early Cardiopulmonary Resuscitation
The next link in the chain is early initiation of CPR, preferably by bystanders. One way to ensure initiation of early CPR is to educate and train a “critical mass” of the general population. The effectiveness of such training has varied widely. In Seattle, approximately 50% of the population has been trained to perform CPR (72), whereas in Minneapolis only 23% of adults surveyed have received such training (94). In smaller cities and in less affluent areas, the number of trained citizens is often much lower. What percentage of adults needs to be trained in CPR to provide reasonable protection in the community is difficult to determine with certainty. As a rule of thumb, the American Heart Association recommends that at least 20% of the adult population should be trained in basic CPR to reduce mortality from out-of-hospital cardiac arrest (95). It is also important to train a critical mass of in-hospital personnel to ensure that proper CPR can be performed on all cardiac arrest victims in all areas of the hospital while awaiting arrival of “code team” personnel.
Virtually all studies have shown that initiation of bystander CPR within 4 minutes of the patient’s collapse results in up to a 12-fold improvement in the odds for survival (90). The mechanism by which early CPR improves outcome is unclear, but may be due to CPR’s ability to keep coarse ventricular fibrillation from degenerating to asystole for a few extra minutes until rescuers arrive. Recent evidence suggests that a brief period (90 seconds to 2 minutes) of CPR actually improves the odds of successful defibrillation if more than several minutes elapses before defibrillation can be provided (59,60).
Early Defibrillation
The rationale for the use of early defibrillation stems from four observations: (a) ventricular tachyarrhythmias are the most common cause of sudden out-of-hospital cardiac arrest in adults; (b) defibrillation is the most effective treatment for pulseless ventricular tachyarrhythmias; (c) the effectiveness of defibrillation diminishes rapidly over time; and (d) unless treated promptly, ventricular fibrillation becomes less coarse and eventually converts to the less treatable rhythm of fine ventricular fibrillation or asystole.
The best outcomes from sudden, arrhythmic cardiac arrest in adults have been reported from cardiac rehabilitation programs, in which defibrillation can be performed within the first minute or two. In such “ideal” settings, 85% to 90% of patients are resuscitated and can be returned to their prearrest neurologic status (96,97,98). Survival from out-of-hospital cardiac arrest treated by EMS personnel has been considerably lower, averaging 15% or 20% or less with a maximum survival of 30%, depending on the EMS system configuration (90).
The best survival is attained in EMS systems that can provide early defibrillation to a large percentage of patients. In most cases, this is most cost-effectively accomplished by a “tiered response” system in which large numbers of rapid “first-response” firefighters or emergency medical technicians (EMTs) are trained and equipped to provide first aid, CPR, and early defibrillation using an automated external defibrillator (AED).
More novel strategies have also been tried to increase the availability of rapid defibrillation in the hospital and in the community at large. Kaye et al. pioneered the use of AEDs by non–intensive care nurses in an in-hospital setting (99,100). There has also been an increasing effort to place AEDs in high-risk public locations for use by trained laypersons.
Early Advanced Cardiac Life Support
Physicians provide prehospital ACLS by staffing specially equipped ambulances in many countries (e.g., western Europe, Scandinavia, Canada). In the United States, “intermediate”-level EMTs or paramedics provide most prehospital ACLS intervention (e.g., defibrillation or synchronized cardioversion, endotracheal intubation, intravenous fluid therapy, drug administration). Intermediate EMTs (often called cardiac technicians) typically receive several hundred hours of training; paramedics usually receive 1,000 or more hours. Adding field ACLS capability appears to favorably affect survival of out-of-hospital cardiac arrest.
The Algorithm Approach to Resuscitation
The American Heart Association’s Advanced Cardiac Life Support (ACLS) algorithms provide a framework for dealing with life-threatening cardiopulmonary emergencies in a logical sequence (101,102). There are sections of the algorithm for initiating CPR and for treating patients with ventricular fibrillation or pulseless ventricular tachycardia, bradycardia, asystole, and pulseless electrical activity (PEA), which was formerly known as electromechanical dissociation (EMD). However, the algorithms are not appropriate for every clinical circumstance, and the team leader must adapt them and the general ACLS principles when necessary. Resuscitation team leaders are encouraged to function as “thinking cooks” when applying these cookbook procedures.
Ventricular Fibrillation or Pulseless Ventricular Tachycardia
Electrical countershock is the treatment of choice for ventricular fibrillation and pulseless ventricular tachycardia (VT). If an initial countershock, intubation, and epinephrine, fail to terminate the arrhythmia (refractory ventricular fibrillation or VT) or if, as in many cases, the arrhythmia rapidly recurs (recurrent ventricular fibrillation or VT), antiarrhythmic drug therapy should be considered (101). There is increasing evidence that biphasic defibrillation waveforms using lower energy (typically 125 to 175 joules) defibrillate successfully and are at least as effective as higher-energy, escalating monophasic waveforms (103,104,105,106,107). The American Heart Association now considers biphasic waveforms or a single 360 J monophasic shock to be preferable to the traditional escalating monophasic waveforms (108,109). Rescuers should follow the Food and Drug Administration (FDA)-approved manufacturer recommendations for energy dosing when using biphasic defibrillator devices.
Pulseless Electrical Activity
Pulseless electrical activity is present when there is organized electrical activity on the electrocardiogram but no effective circulation. There are potentially many underlying causes, but the most common denominator may involve myocardial ischemia and dysfunction due to intramyocardial increases in carbon dioxide (110). Prognosis is generally poor unless a discrete and treatable etiology for PEA can be discerned and corrected. Because of the poor prognosis when a correctable etiology cannot be defined, efforts should be directed toward detecting causes such as hypovolemia, tension pneumothorax, and pericardial tamponade (101). Normal saline or Ringer’s lactate solution should be infused rapidly if there is evidence of hypovolemia. Suspected pneumothorax or pericardial tamponade should be confirmed by needle aspiration of the chest or pericardium, respectively. If confirmed, more definitive surgical management (chest tube or thoracotomy) is usually required.
Scrutiny of the neck veins may be helpful in attempting to define an etiology for PEA. Most patients with cardiac arrest have high right-sided filling pressures and distended neck
veins. When neck veins are not visible in this setting and PEA is present, hypovolemia should be suspected. In the “trauma” cardiac arrest victim (such as a patient with a gunshot wound of the chest), however, prominent neck veins should lead to the suspicion of pericardial tamponade or tension pneumothorax (111).
veins. When neck veins are not visible in this setting and PEA is present, hypovolemia should be suspected. In the “trauma” cardiac arrest victim (such as a patient with a gunshot wound of the chest), however, prominent neck veins should lead to the suspicion of pericardial tamponade or tension pneumothorax (111).
General measures such as (a) support of ventilation, (b) properly performed closed-chest compression, and (c) frequent doses of epinephrine to maintain arterial perfusion pressure and coronary and cerebral perfusion are recommended for treatment of PEA (101). Bradycardia may be treated with atropine. Although catecholamines are frequently given, there are no data to suggest a specific benefit (other than improvement in coronary and cerebral blood flow during closed-chest compression). Calcium chloride has not been shown to affect clinical survival in controlled trials (112,113). In addition, the pathologically high serum calcium levels caused by its administration (114,115) may exacerbate reperfusion injury (116).
If penetrating cardiac trauma is present, open chest massage can be live saving (117). In other settings, open-chest massage is rarely of value, partly because it is usually initiated late after the onset of the arrest (118). Prehospital trauma victims who are pulseless and have asystole or agonal electrical cardiac activity (heart rate <40 beats per minute) have such a poor prognosis that some authors feel that they should be pronounced dead at the scene of injury (119).
Bradyasystole
Bradyasystole is one of the most common and least understood problems that can occur during resuscitation. For purposes of definition, bradyasystole refers to a cardiac rhythm that has a ventricular rate below 60 beats per minute in adults and/or periods of absent heart rhythm (asystole). Bradyasystolic states are clinical situations during which bradyasystole is the dominant heart rhythm.
Survival is poor (generally 1% to 3% or less) regardless of therapy for patients who present with bradyasystole. It is always important to exclude disconnection of a lead or monitor electrode prior to concluding that a “flat line” is the patient’s rhythm. Because some patients with a “flat line” may have ventricular fibrillation (a rhythm more amenable to treatment) masquerading as asystole (120), the monitor lead configuration should be quickly switched to a second lead to confirm the diagnosis before treatment (101). Other general measures recommended for the treatment of bradyasystole include support of ventilation, properly performed closed-chest compression, and frequent doses of epinephrine to maintain arterial perfusion pressure and coronary and cerebral perfusion (101).
Treatment with atropine sulfate may improve outcome in patients with bradyasystolic cardiac arrest that is due to excessive vagal stimulation, but atropine is less effective when asystole or pulseless idioventricular rhythms are the result of prolonged ischemia or mechanical injury in the myocardium (121).
For patients with bradyasystolic cardiac arrest, a 1-mg dose of atropine is administered intravenously (IV) and is repeated every 3 to 5 minutes if asystole persists. Three milligrams (0.04 mg/kg) given IV is a fully vagolytic dose in most patients (122). The administration of a total vagolytic dose of atropine should be reserved for patients with bradyasystolic cardiac arrest. Endotracheal atropine produces a rapid onset of action similar to that observed with IV injection. The recommended adult dose of atropine for endotracheal administration is 1.0 to 2.0 mg diluted in 10 mL of sterile water or normal saline.
Pacing (transvenous, transthoracic, or transcutaneous) rarely influences survival in the unwitnessed cardiac arrest patient who is initially found with asystole or bradycardia without a pulse (123,124,125). However, pacing is extremely useful for bradycardic patients with a pulse and in selected patients in whom a pacemaker can be placed immediately after the development of the conduction disturbance (124,125). In such cases, a precordial thump can also stimulate ventricular complexes and a pulse (“fist pacing”) (126).
Endogenous adenosine released during myocardial hypoxia and ischemia relaxes vascular smooth muscle, decreases atrial and ventricular contractility, depresses pacemaker automaticity, and impairs atrioventricular conduction (127). The cellular electrophysiologic effects of adenosine can be competitively antagonized by methylxanthines but not by atropine. A specific adenosine antagonist (BW-A1433U) has been shown to reverse and prevent postdefibrillation bradyasystole and hemodynamic depression in a domestic pig model (128).
Aminophylline, a competitive nonspecific adenosine antagonist, has been shown to restore cardiac electrical activity within 30 seconds in 12 of 15 in-hospital bradyasystolic cardiac arrest patients who were refractory to atropine and epinephrine (129). Other anecdotal case reports seem to indicate that adenosine blockade may restore normal sinus rhythm in some bradyasystole patients who do not respond to conventional therapy (130). In a relatively small randomized, prospective clinical trial on adults with asystole refractory to epinephrine and atropine there was a trend towards improved ROSC in patients who received aminophylline versus placebo, but the results did not reach statistical significance (131). Forty-five patients were assigned to the placebo group and 37 received aminophylline. Nine of 45 controls (20%; 95% confidence interval [CI] 10% to 35%) achieved ROSC compared to 10 of 37 (27%; 95% CI 14% to 44%) in the aminophylline group. Although further clinical research will be necessary to determine the potential value of adenosine blockade for bradyasystolic cardiac arrest, it is clear that these agents should not be used when ventricular fibrillation is present because they may make it more difficult to terminate this arrhythmia (132).
Physiologic Monitoring during Resuscitation
Real-time hemodynamic data can provide the team leader with a sound physiologic basis for altering the approach to clinical resuscitation when the standard algorithms either fail or do not apply.
Arterial Pressure Measurement During Cardiopulmonary Resuscitation
Maintenance of both the systolic and diastolic arterial pressure is vital during CPR. Because flow to most vital organs (except the heart) occurs during systole, a minimal systolic arterial pressure of 50 to 60 mm Hg is usually required to resist arteriolar collapse (133). Diastolic pressure is also important because it is a critical determinant of the coronary perfusion pressure (CPP = aortic diastolic – right atrial pressure) during CPR. CPP is one of the best hemodynamic predictors of ROSC in both animal models (20,134,135,136,137,138,139,140,141,142,143,144) and humans (145). A minimal threshold gradient for CPP of approximately 15 mm Hg (usually corresponding to an aortic diastolic pressure of 30 to 40 mm Hg) provides a level of myocardial blood flow that is necessary to meet the metabolic needs of the arrested myocardium (146) and to achieve ROSC (145).
A cuff manometer can be used to estimate the systolic arterial pressure during CPR by palpating the brachial artery or listening over it for flow with a peripheral Doppler device
(147). More accurate, invasive measurements of systolic and diastolic pressure can be obtained by inserting an arterial line into the radial, brachial, or femoral artery (26,148,149,150,151,152,153,154,155). Table 69.1 summarizes recent published invasive arterial pressure measurements during resuscitation in adult humans. Conventional CPR generates systolic, diastolic, and coronary perfusion pressures that are inadequate to sustain blood flow and function in vital organs (e.g., the brain). These measurements explain why cardiac arrest patients are rarely conscious during CPR.
(147). More accurate, invasive measurements of systolic and diastolic pressure can be obtained by inserting an arterial line into the radial, brachial, or femoral artery (26,148,149,150,151,152,153,154,155). Table 69.1 summarizes recent published invasive arterial pressure measurements during resuscitation in adult humans. Conventional CPR generates systolic, diastolic, and coronary perfusion pressures that are inadequate to sustain blood flow and function in vital organs (e.g., the brain). These measurements explain why cardiac arrest patients are rarely conscious during CPR.
TABLE 69.1 Arterial Pressure Measurements during Cardiopulmonary Resuscitation in Humans | |||||||||||||||||||||||||||||||||||||||||||||||||||||||||||
---|---|---|---|---|---|---|---|---|---|---|---|---|---|---|---|---|---|---|---|---|---|---|---|---|---|---|---|---|---|---|---|---|---|---|---|---|---|---|---|---|---|---|---|---|---|---|---|---|---|---|---|---|---|---|---|---|---|---|---|
|
Pulmonary Artery Catheter Recordings
Generation of a satisfactory arterial pressure is a prerequisite for adequate flow to vital organs; however, the presence of a reasonable arterial pressure does not ensure that there will be sufficient flow to meet metabolic needs. Pulmonary artery catheters are inserted infrequently during resuscitation because they are time consuming to set up and technically difficult to position. The low flows generated by CPR do not favor ready passage of the balloon-flotation catheter through the right heart. In addition, it is difficult to determine the catheter tip’s position from pressure recordings because the pressures in the intracardiac chambers and great vessels are similar to each other during closed-chest CPR (25,156,157,158,159,160). Fluoroscopy is of little value during CPR, even if it is available, because it is not easy to continue chest compression impeded by the machine’s C-arm. Transesophageal echocardiography (TEE) offers the greatest promise as a means of visualizing and helping to position intracardiac catheters successfully during CPR.
Despite these technical limitations, there have been several published reports of intracardiac pressure and flow measurements in humans who were already being monitored invasively in an intensive care unit at the time of their cardiac arrest (161,162,163,164). Conventional, closed-chest CPR in adult humans produces a hemodynamic state resembling profound cardiogenic shock, with a low systemic arterial pressure, markedly reduced cardiac output, and high intravascular filling pressures. Hemodynamic monitoring post-CPR may be useful for managing selected patients who survive resuscitation, but the risks, potential benefits, and cost implications must be weighed on a case-by-case basis (164).
End-Tidal Carbon Dioxide Monitoring During Resuscitation
The percentage of carbon dioxide contained in the last few milliliters of gas exhaled from the lungs with each breath is known as the end-tidal carbon dioxide concentration (PetCO2) (165,166). It can be measured by passing an infrared beam through exhaled gases and measuring the decrease in voltage output from a photoelectric cell calibrated to the wavelength at which carbon dioxide absorbs light. It can also be estimated by attaching an inexpensive disposable plastic, colorimetric device in-line between the endotracheal (ET) tube and the ventilation device. Color indicators on the device change hue as the exhaled gases pass through the monitor’s pH-sensitive membranes. The membrane’s color can be compared with the adjacent color chart on the device, thus indicating the percentage PetCO2.
Two important terms need to be defined with respect to end-tidal carbon dioxide monitoring. Capnography refers to any technology that displays and/or prints the actual capnographic waveform. If an unmistakable capnographic waveform is detected when a capnographic probe is attached to a patient who is being ventilated, then the airway device is unequivocally ventilating the lungs. Capnometry refers to the measurement or estimation of the end-tidal carbon dioxide concentration. It can be displayed in analogue or digital format, or it can be approximated using a colorimetric detection device. Capnometry often provides useful information on the anatomic location of an airway device (e.g., ET tube); however, it does not provide the degree of definitive certainty that the presence of a capnographic waveform does.
During normal respiration and circulation, the PetCO2 averages 4% to 5%. Two units of measure are popularly used in reporting the PetCO2: percent and mm Hg (1% is approximately 7 mm Hg). There is a logarithmic relationship between the PetCO2 and the cardiac output (167). At normal or elevated levels of cardiac output, ventilation is the rate-limiting factor responsible for eliminating the large amount of carbon dioxide traversing the pulmonary circuit (e.g., hyperventilation lowers, and hypoventilation raises, the PetCO2). In this range, if blood flow and arterial pressure are kept reasonably constant, the PetCO2 closely approximates arterial carbon dioxide tension (PaCO2) and can be used as a “real-time” guide to the
adequacy of ventilation (the PetCO2 actually lags behind real-time events by a few seconds, the amount of time it takes for exhaled gas to be drawn into the machine and analyzed) (168).
adequacy of ventilation (the PetCO2 actually lags behind real-time events by a few seconds, the amount of time it takes for exhaled gas to be drawn into the machine and analyzed) (168).
The PetCO2 can also be used to confirm ET airway placement, particularly in the non–cardiac arrest patient who has a pulse and an adequate blood pressure (where the sensitivity and specificity of the PetCO2 for detecting correct ET tube placement approach 100% and 90%, respectively) (154,169,170,171,172,173). The ET tube cuff should be inflated when the PetCO2-monitoring device is attached to ensure that the gas being sampled is from the structure (i.e., trachea or esophagus) in which the tube resides. Ventilation through an ET tube that has been properly inserted in the trachea yields a PetCO2 of 4% to 5% in a patient with a normal cardiac output and no significant ventilation/perfusion gradient; ventilation through an ET tube that has been inadvertently inserted into the esophagus results in a PetCO2 of less than 0.5%. The only important exception occurs when the ET tube is inserted into the esophagus of a patient who has recently ingested a carbonated beverage (e.g., beer, soda pop). In such a case, the initial PetCO2 will appear to be normal or high on the first few ventilations but will typically decrease rapidly to less than 0.5% by the sixth or seventh ventilation (174).
At low levels of cardiac output (below approximately 50% of normal in animal models), ventilation has much less effect on the PetCO2. Instead, the pulmonary blood flow (and the subsequent ventilation/perfusion mismatch) is the rate-limiting factor determining the PetCO2. If ventilation is kept relatively constant in this range, an increase or a decrease in the cardiac output will usually be reflected by a rise or fall in the PetCO2, respectively.
During CPR the cardiac output falls typically to between one fourth and one third of normal (162,175,176,177). The PetCO2 also decreases to between one fourth and one third of normal as the reduced cardiac output and pulmonary blood flow present less blood and carbon dioxide to the lung for exchange with oxygen in the pulmonary capillary (178
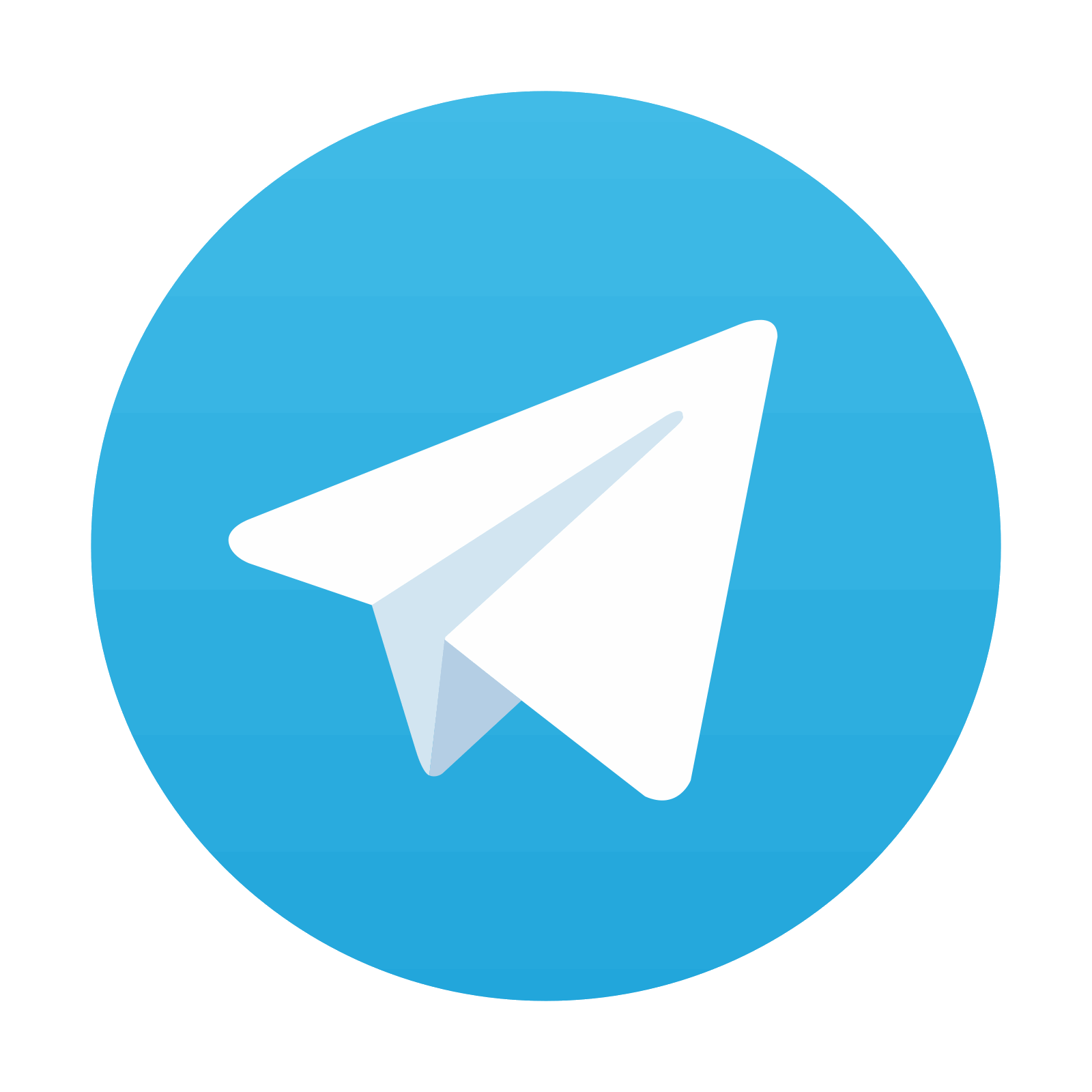
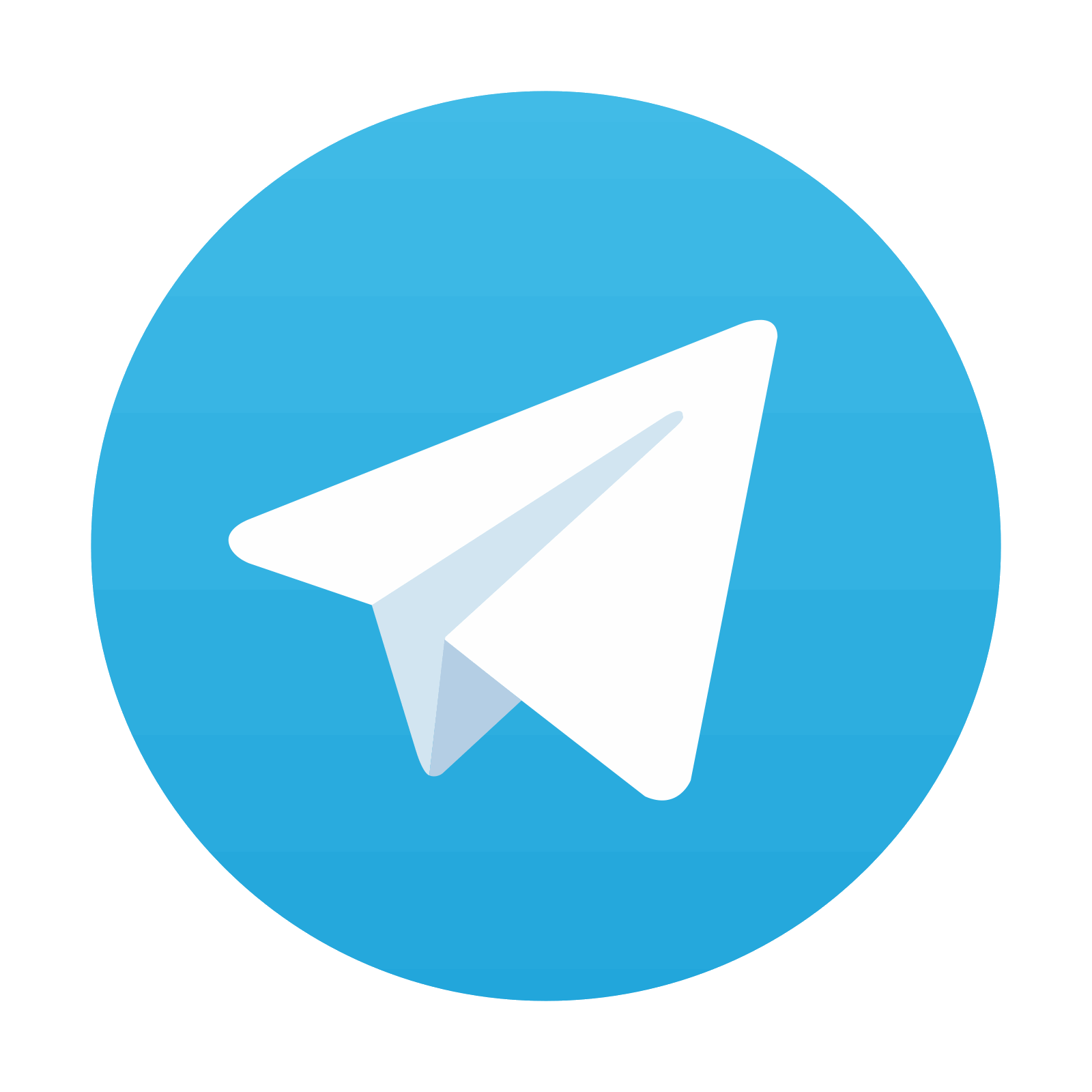
Stay updated, free articles. Join our Telegram channel
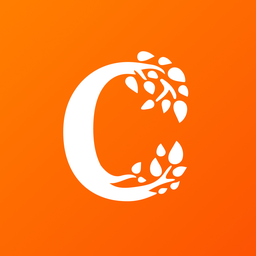
Full access? Get Clinical Tree
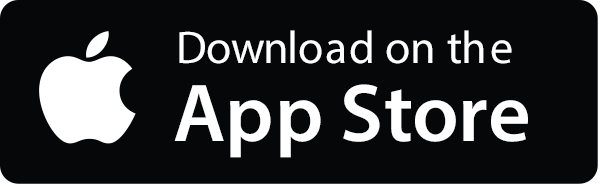
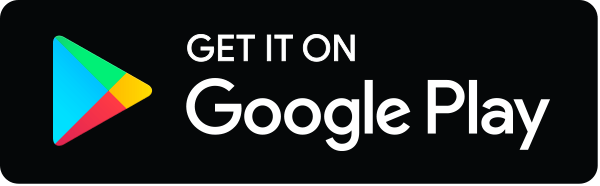
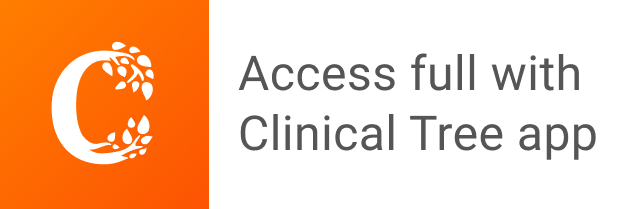