Fig. 16.1
Pressure flow curve (water) Medtronic DLP 77108 (Provided by the manufacturer)
We can represent these data by a parabolic fit:
In the example above, we obtain:


In order to obtain the values for blood with a given hematocrit and temperature, we need to rescale the coefficients with ratios of density and dynamic viscosity:
Calculate density and viscosity for water and blood
Blood characteristics during cardiopulmonary bypass
![$$ \begin{array}{l}\mathrm{Hct}:=25\%\kern1.25em \mathrm{Hematocrit}\left[\%\right]\hfill \\ {}{T}_{\mathrm{blood}}:=32\kern1.25em \mathrm{Arterial}\kern0.5em \mathrm{blood}\kern0.5em \mathrm{temperature}\left[\circ \mathrm{C}\right]\hfill \end{array} $$](https://i0.wp.com/thoracickey.com/wp-content/uploads/2017/09/A322400_1_En_16_Chapter_Equc.gif?w=960)
Calculate ratios
What is the pressure drop over a 77108 DLP cannula during CPB at a blood flow of 0.8 L/min?
Assessing access of the venous side is more complex due to more stringent boundary conditions. In the normal circulation, venous return toward the heart is governed by the pressure difference between the mean circulatory filling pressure and the pressure in the right atrium.


![$$ \begin{array}{l}\mathrm{Hct}:=25\%\kern1.25em \mathrm{Hematocrit}\left[\%\right]\hfill \\ {}{T}_{\mathrm{blood}}:=32\kern1.25em \mathrm{Arterial}\kern0.5em \mathrm{blood}\kern0.5em \mathrm{temperature}\left[\circ \mathrm{C}\right]\hfill \end{array} $$](https://i0.wp.com/thoracickey.com/wp-content/uploads/2017/09/A322400_1_En_16_Chapter_Equc.gif?w=960)
![$$ \begin{array}{l}{r}_{\mathrm{blood}}=1.09\frac{\mathrm{gm}}{{\mathrm{cm}}^3}\cdot \mathrm{Hct}+1.035\frac{\mathrm{gm}}{{\mathrm{cm}}^3}\cdot \left(1-\mathrm{Hct}\right)\hfill \\ {}{r}_{\mathrm{blood}}=1.049\times {10}^3\frac{\mathrm{kg}}{{\mathrm{m}}^3}\kern0.85em \mathrm{Blood}\kern0.5em \mathrm{density}\kern0.5em \mathrm{during}\kern0.5em \mathrm{CPB}\hfill \\ {}{h}_{\mathrm{plasma}}=\frac{ \exp \left[-5.64+\frac{1800}{\left({T}_{\mathrm{blood}}+273\right)}\right]}{100}\cdot \mathrm{poise}\hfill \\ {}{h}_{\mathrm{blood}}={h}_{\mathrm{plasma}}\cdot \exp \left(2.31\cdot \mathrm{Hct}\right)\kern0.85em {h}_{\mathrm{blood}}=2.314\cdot \mathrm{cpoise}\hfill \end{array} $$](https://i0.wp.com/thoracickey.com/wp-content/uploads/2017/09/A322400_1_En_16_Chapter_Equd.gif?w=960)


This pressure difference is approximately 7 mmHg (Guyton et al. 1954, 1957, 1962). When venous access is established, the wide, low-resistance, collapsible blood vessels are connected with smaller-diameter, stiff, artificial conduits of known physical characteristics. Because of the smaller diameters of both the venous line and the venous cannula, higher pressure differences are needed to obtain optimal venous drainage (Galletti and Brecher 1962). The necessary pressure difference to achieve optimal drainage will depend on tubing diameter, tubing length, and blood viscosity (Ni et al. 2001). The latter in its turn will be dependent on both hematocrit and temperature. In order to overcome the combined pressure drop of both venous cannula and venous line, a negative pressure is applied by using gravity (siphon) or assisted venous drainage. When applying negative pressure, venous drainage will first increase linearly with the increase in negative pressure, while the resistance will remain more or less constant. However, at a certain point, a further increase in pressure will partially collapse the vein, with no further increase in blood flow, and, therefore, resistance will start to increase (Galletti and Brecher 1962). Correct choice of a venous cannula is critical as it will represent the smallest diameter and, thus, the highest resistance. Reducing the diameter by 50 % will reduce flow to 1/16th of the original flow. Also, the length of this smallest diameter is important, as doubling the length of the cannula will decrease flow by 50 %. Another point of interest is the ratio between the diameter of the vein and the cannula. According to Galetti, this ratio should be around 0.5 in order to avoid collapse of the vein around the side holes of the cannula (Galletti and Brecher 1962). Although the diameter ratio is important, also the design of the cannula and cannula tip (De Somer et al. 2002) will influence the drainage efficiency.
In children vacuum-assisted venous drainage (VAVD) is becoming more and more the standard. This technique applies vacuum on the venous reservoir in order to increase the pressure differential (Durandy and Hulin 2006; Durandy 2009a, b). However, it should not be used as a solution for correcting improper cannula position. If VAVD is used under such conditions, one mainly will increase resistance in the cannula. At the same time, a higher blood velocity will be generated over those openings of the cannula which are not blocked by the improper position. Finally, this will increase shear stress and lead to an increase in hemolysis. Before applying any form of assisted venous drainage, one should check proper cannula placement. After this check, one can use assisted venous drainage in cases where one wants to reduce priming volume by placing the oxygenator at the same height as the patient or by using a smaller-diameter venous line or by combining both strategies (Pappalardo et al. 2007).
The reported vacuum that should be applied for optimal drainage lies between −30 and −80 mmHg. The absolute value will depend upon where this negative pressure is measured in the circuit. The purpose of measuring the pressure is to estimate the pressure at the cannula tip as this will give us information with respect to risk for vein collapse. Vein collapse will occur once the negative pressure at the cannula tip exceeds −4 mmHg. Unfortunately, the pressure at the cannula tip is difficult to obtain in clinical practice so most perfusionists measure the pressure somewhere between the cannula and the reservoir top. As a result, the obtained pressure value is the sum of the resistance in the cannula and the venous line between the measurement point and the cannula tip. The latter might explain the large differences in reported values. This is illustrated in Table 16.1, which shows the impact on blood velocity and required pressure difference when 3/16 in. tubing or 1/4 in. tubing is used for obtaining a venous drainage of 1 L/min.
Table 16.1
Impact of tubing diameter on venous line pressure drop
Tubing diameter [inch] | 3/16 | 1/4 |
---|---|---|
Blood flow [L/min] | 1 | 1 |
Pressure difference [mmHg] | 51 | 11 |
Velocity [cm/s] | 94 | 53 |
Reynolds number | 2019 | 1514 |
Wall shear stress [dynes/cm2] | 54 | 15 |
In general, assisted venous drainage is helpful in all cases where siphon drainage alone is insufficient due to high resistances in the venous cannula and venous line and in cases where venous pressure remains high despite proper cannula position and in those cases where the operative field is not dry (Murai et al. 2005).
Optimizing arterial and venous vascular access is mandatory as it will determine the maximum blood flow that can be obtained and thus oxygen delivery to the organs. Malposition of a cannula can obstruct cerebral blood supply or cause a preferential flow into the descending aorta leading to an inappropriate oxygen supply to the brain. Alternatively obstruction of the superior vena caval cannula may decrease cerebral venous drainage and potentially lead to brain dysfunction. Nowadays, many of these problems can be detected early in time by routine use of cerebral oxygenation by near-infrared spectroscopy (NIRS) (Gottlieb et al. 2006; Ginther et al. 2011; Redlin et al. 2011).
Tubing
The tubing in the CPB circuit interconnects all of the main components of the circuit. A variety of polymers can be used for the manufacture of tubing, but most are made of PVC with exception of the tubing used in the pump boot which is often silicone. The length and size of the tubing will have a major impact on volume, shear stress, and pressure drop (Table 16.2), and the clinician will have to make a choice based upon the clinical conditions.
Table 16.2
Characteristics of different pediatric tubings
Tubing diameter [inch] | 1/8 | 3/16 | 1/4 | 3/8 |
---|---|---|---|---|
Volume [mL/m] | 8 | 18 | 32 | 71 |
Pressure difference [mmHg/L] | 234 | 54 | 15 | 5 |
Velocity [cm/s] | 210 | 94 | 53 | 23 |
Reynolds number | 3028 | 2019 | 1514 | 1009 |
Wall shear stress [dynes/cm2] | 247 | 54 | 15 | 6 |
Blood Pumps
From an engineering perspective, pumps can be classified into two main categories: displacement pumps and rotary pumps. The energy in displacement pumps is characterized by periodic volumetric changes of a working space. A typical displacement pump is the roller pump. The principle of operation of this pump is that two rollers, placed opposite to each other, “roll” the blood through a piece of tubing. When the pump completely occludes the tubing, the pump is capable of generating both positive and negative pressures, and therefore it can be also used as blood aspirating pump. Because of its working principle, a roller pump is relatively independent of factors such as resistance and hydrostatic pressure head, which are encountered in the average CPB circuit. The output of an occlusive roller pump depends upon two main variables: the number of revolutions per minute of the pump head and the internal diameter and length of the tubing held in the pump head:
where RPM = revolutions per minute.

A disadvantage of roller pumps is spallation (Briceno and Runge 1992; Peek et al. 2000). Due to the continuous compression of the tubing by the roller, the polymer of the tubing starts to weaken and to erode, resulting in the generation of small particles (Briceno and Runge 1992; Kim and Yoon 1998; Peek et al. 2000). In order to control spallation, it is advocated to use a dynamic occlusion setting of the pump rollers (Tamari et al. 1997). Due to the high resistances encountered in neonatal and pediatric CPB circuits, roller pumps remain the first choice. In larger children or young adults, one might prefer a rotary pump and more specific a centrifugal pump. Centrifugal pumps operate on the principle of moving fluid by creating a pressure gradient between the inlet and the outlet of the pump. The pressure gradient results from the creation of a vortex by the rotation of the pump head. The rotating motion creates an area of low pressure in the center and an area of high pressure on the sides. The resulting rate of blood flow will depend upon the pressure gradient and the resistance at the outlet of the pump. The latter is a function of two variables: the CPB circuit (oxygenator, filter, tubing, arterial cannula) and the systemic vascular resistance of the patient. Because the flow produced by a centrifugal pump directly depends on the pressure that the centrifugal pump generates, a centrifugal pump is called a pressure pump. In contrast to a roller pump, a centrifugal pump is afterload dependent and thus influenced by changes in resistance in both the circuit and the patient. For this reason a centrifugal pump should always be used in conjunction with a flow meter. Although a centrifugal pump, due to its non-occlusive working principle, has no spallation, high resistances after the pump may lead to high shear stresses and hot spots inside the pump head (Araki et al. 1995a, b; Ganushchak et al. 2006).
Oxygenator
The oxygenator is without doubt the most important component in the CPB circuit. It is not only responsible for exchanging oxygen and carbon dioxide but also for the administration of volatile anesthetics. The oxygenator comprises an integrated heat exchanger that allows cooling and warming of the patient. A heat exchanger is indispensible as some extensive repairs may require hypothermia and/or deep hypothermic circulatory arrest (DHCA). Most recent oxygenators are now available with an integrated filter thus avoiding the need for a separate arterial line filter (Lin et al. 2012; Ginther et al. 2013). In pediatric surgery most centers use an open venous reservoir with integrated cardiotomy. The latter filters and defoams blood aspirated from the surgical field. The main reason for choosing open systems lays in the fact that open systems allow assisted venous drainage which is helpful in optimizing venous drainage and in reducing priming volume (Durandy 2013, 2015).
Nowadays, exclusively extraluminal hollow fiber membrane oxygenators are used. For neonatal and pediatric usage, several sizes are available. The final decision which to use is usually made based upon priming volume, surface area, rated blood flow, and available connections all in relation to the size of the patient and the type of surgical repair. Table 16.3 shows the characteristics of some neonatal and pediatric oxygenators. Originally the reference flow of a given oxygenator was defined by the Association for the Advancement of Medical Instrumentation (AAMI) as the flow rate at which normothermic whole blood having a hemoglobin content of 120 g/L, a base excess of 0, and a venous saturation of 65 % will increase its oxygen content by 45 mL oxygen per liter blood. This proposed value offered sufficient safety in acyanotic children but could pose a problem in cyanotic children that are often presented with much lower venous saturation. For this reason, contemporary pediatric oxygenators easily can have oxygen transfers up to 75 mL/L at the nominal maximum flow given by the manufacturer. As a result the reference flow (AAMI conditions) can be much higher (Table 16.3) than the recommended flow. Based upon this characteristic, one could use a smaller oxygenator, with the resulting lower hemodilution and contact activation, in selected cases (Durandy 2010a).
Table 16.3
Characteristics of contemporary neonatal and pediatric oxygenators
Oxygenator | Membrane surface area [m2] | Membrane material | Maximum blood flow [L/min] | Reference blood flow [L/min] | Heat exchanger surface area [m2] |
---|---|---|---|---|---|
Terumo FX05 | 0.5 | PP | 1.5 | 2.5 | 0.035 |
LivaNova D100a | 0.22 | PP | 0.7 | 1 | 0.03 |
LivaNova D101a | 0.61 | PP | 2.5 | 3.5 | 0.06 |
Maquet Neonatal Quadrox-i | 0.38 | PP | 1.5 | N/A | 0.07 |
Maquet Pediatric Quadrox-i | 0.8 | PP | 2.8 | N/A | 0.15 |
Medtronic Pixiea | 0.67 | PP | 2 | N/A | N/A |
Medos Hilite 1000a | 0.39 | PP | 1 | N/A | 0.074 |
Medos Hilite 2800a | 0.8 | PP | 2.8 | N/A | 0.16 |
Priming and Hemodilution
The total priming volume of a CPB circuit is determined by the components selected (De Somer et al. 1996b). It is important to select a smaller oxygenator that will function close to its maximal capacity for flow rather than selecting a large oxygenator that will function toward its lower level. However, independent of the choice of oxygenator, its priming volume will be fixed. The priming volume taken by the tubing, on the other hand, is determined by its length and diameter and mainly controlled by the surgical team (Ni et al. 2001). The total amount of priming volume is important as it will determine the dilution of the blood components. However, also the composition of the priming fluid is a point of consideration. Excessive dilution of blood coagulation factors below 45 % should be prevented by using fresh frozen plasma in the priming solution (Brauer et al. 2013). This is especially important in cyanotic children as they have in general a lower plasma volume or in children with complex repairs (Pouard and Bojan 2013). As many institutions do not routinely screen coagulation factors before cardiac surgery, often fibrinogen concentration is used as a reference, keeping its concentration above 1 g/L. Huge differences in target hemoglobin during CPB are reported going from 50 to 100 g/dL (Nicolas et al. 1994; Gruber et al. 1999). Due to a lack of sufficient randomized prospective studies (Wilkinson et al. 2014), it is still unclear what is the optimal hemoglobin concentration during CPB. But one should not focus alone on hemoglobin concentration as final oxygen delivery (DO2) will be dependent on both hemoglobin concentration and pump flow. As a consequence one can tolerate lower hemoglobin concentrations as long as vascular access allows for high pump flows, but when anatomical limitations restrict vascular access, a higher hemoglobin concentration might be desirable. This can be easily demonstrated by the following example: if we consider a minimum DO2 of 300 mL/min/m2 in a child with a body surface of 0.22 m2, then the required blood flow would be 490 mL/min at a hemoglobin of 100 g/L, but we would need a blood flow of 1970 mL/min at a hemoglobin of 50 g/L to achieve the target DO2. It is quite obvious that the latter is hardly obtainable.
Prophylactic use of both fresh frozen plasma and packed red cells without arguments is not recommended (Wilkinson et al. 2014; Desborough et al. 2015).
Beside the impact of the priming solution on blood coagulation and oxygen transport, its composition will also affect colloid oncotic pressure. There is evidence that priming with a high-colloid oncotic pressure, by adding albumin, results in less fluid overload by the end of CPB and is advantageous in neonates compared to a priming solution only containing crystalloids (Pouard and Bojan 2013).
Reflection on the composition of the priming volume and composition becomes even more important; now more and more centers prefer normothermic conditions even for complex repairs such as transposition of the great arteries (Durandy 2010b).
In the early days of cardiac surgery, hemodilution was seen as a huge benefit for the cardiac surgical population of that time as it could avoid blood prime. Despite this advantage it became obvious over the years, due to better monitoring techniques and extensive research, that hemodilution has its limits. Hemodilution will have a linear impact, when blood flow is constant, on total oxygen content. Hemodiluting a patient with a normal hematocrit of 40 % to a hematocrit of 20 % will thus decrease the oxygen content per liter blood by 50 %. When this happens in healthy patients, not on CPB bypass, this loss will be compensated by a compensatory increase in cardiac output which is facilitated by the reduced viscosity caused by the hemodilution. During CPB a fixed blood flow per square meter of body surface is used, typically between 2.2 and 3.0 L/min/m2. Maintaining this fixed flow during excessive hemodilution might jeopardize oxygen delivery to the tissue as the physiological compensatory increase in flow is absent.
Another disadvantage of hemodilution is the decrease in viscosity and plasma proteins. The decrease in viscosity leads to a loss in density of capillaries in the microcirculation (Tsai et al. 1998). Research has shown that this negative effect can be in part attenuated by using fluids with a higher viscosity for hemodilution as increasing the viscosity of plasma is directly associated with increased perivascular nitric oxide concentration, an effect related to vasodilatation, and increased perfusion and capillary density compared with the same procedure using fluids with a lower viscosity (Tsai et al. 2005). Although the fluids used in this study had a viscosity much higher than those commercially available, it seems favorable to use priming solutions with a higher viscosity (Manduz et al. 2008). The decrease in plasma proteins will result in a decrease in plasma colloid oncotic pressure which may play an important role in the fluid accumulation often seen after CPB. Tissue edema is secondary to increased capillary permeability caused by the systemic inflammatory response induced by CPB. In neonates the combination of this increased capillary permeability and the decrease of the colloid oncotic pressure seems to worsen the situation (Jonas 2004). Maintaining colloid oncotic pressure during bypass has been linked to decreased myocardial edema (Foglia et al. 1986) and reduced fluid accumulation. The lower fluid accumulation was then associated with a shorter stay in intensive care and a lower mortality (Haneda et al. 1985).
Metabolism During CPB
The primary function of CPB is to maintain the circulation in order to prevent organ dysfunction during and after the surgical repair. Oxygen delivery is one of the most important variables in achieving this goal. Oxygen delivery will depend upon the hemoglobin concentration and the pump flow. In adults it has been demonstrated that there exist a close correlation between the lowest hematocrit on bypass and morbidity (Habib et al. 2003). However, it is questioned whether this is due to the low hematocrit by itself or due to a low DO2 (Ranucci et al. 2005). Recent research showed that maintaining DO2 above 280 mL/min/m2 could reduce the incidence of acute kidney injury from 29.8 to 12.1 %. As this research was done in an adult population at temperatures above 32°C, one could question whether these findings could be extrapolated to a pediatric population. But it clearly demonstrates that when a low hematocrit is targeted during CPB, one will need to maintain higher blood flows. On the other hand, when anatomical limitations limit the size of the vascular access, one should keep the hematocrit higher during CPB.
The microcirculation is the ultimate destination of red blood cells (RBC) to transport oxygen to the tissue cells. Its success defines the primary function of the cardiovascular system. Inside the microcirculation there are two main determinants of oxygen transport to the tissue, convective transport of red blood cells to the capillaries and the passive diffusion of oxygen leaving the RBC to the mitochondria in the cells (Ince 2014). The convective transport is represented by:
where Q = blood flow [mL/min], Hb = hemoglobin concentration [g/mL], cte = [1.34 mL/g], S = amount of oxygen bound to hemoglobin[%], PO2 = partial oxygen tension [mmHg], and k = oxygen solubility [mL/mL].
![$$ {\mathrm{DO}}_2=\left[\left(\mathrm{cte}\cdot \mathrm{Hb}\cdot S\right)+\left({\mathrm{PO}}_2\cdot k\right)\right]\cdot Q $$](https://i0.wp.com/thoracickey.com/wp-content/uploads/2017/09/A322400_1_En_16_Chapter_Equh.gif?w=960)
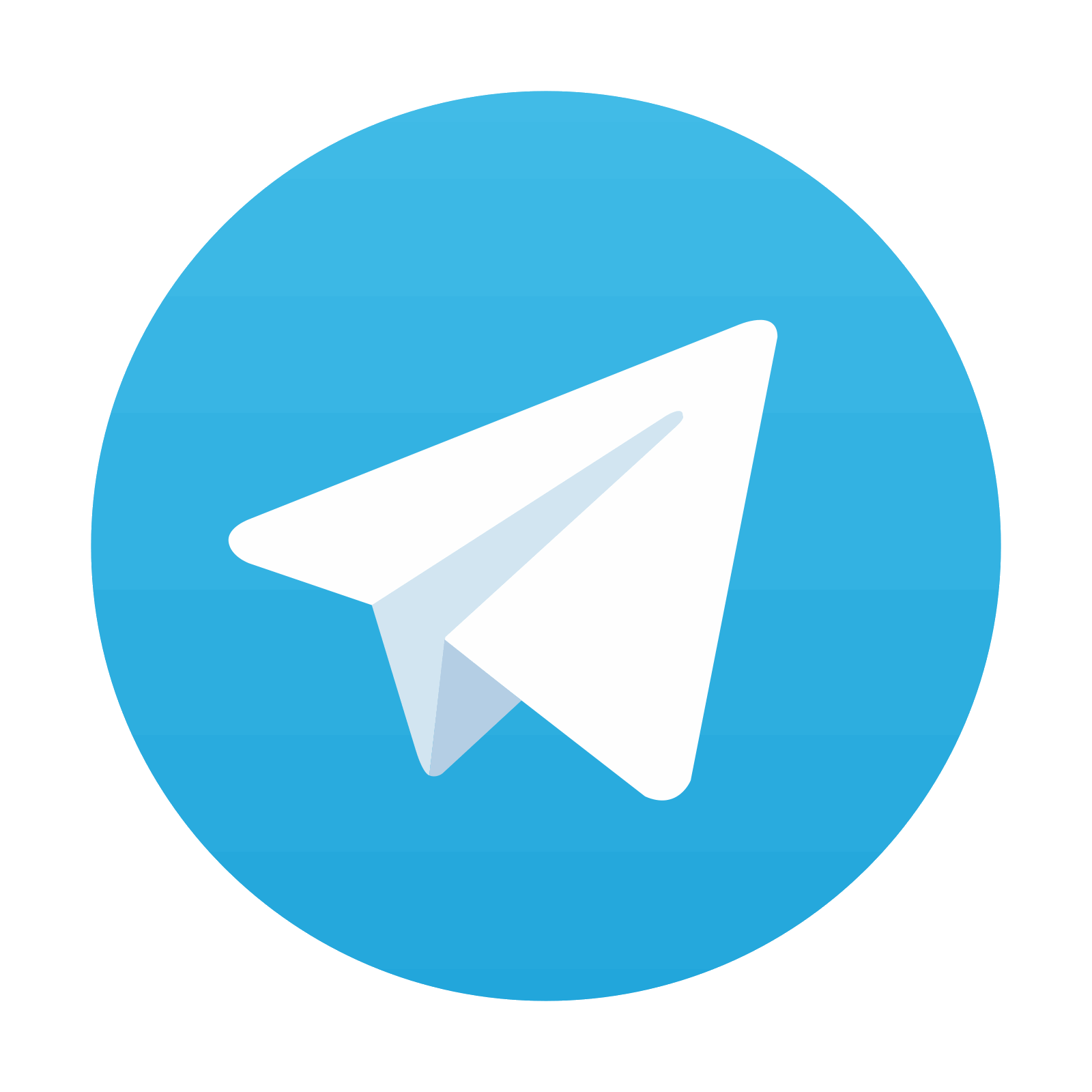
Stay updated, free articles. Join our Telegram channel
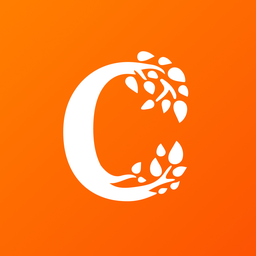
Full access? Get Clinical Tree
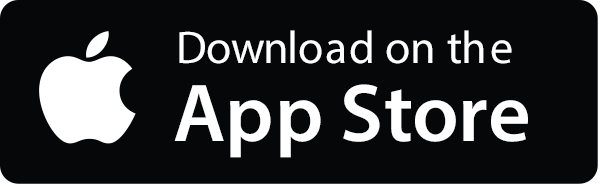
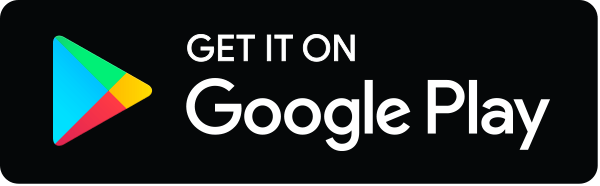