1 Cardiac Electrical Stimulation
Concepts Related to Electrical Stimulation of the Heart
Inductance
Note that the voltage across the inductor is directly proportional to the rate of change of current flowing through the inductor. Cell membrane currents have some of the current- and voltage-versus-time characteristics of an inductance in parallel with a capacitance.1 These inductance-like effects are related to the timing and magnitude of potassium ions moving into and out of the cell.2
Reactance (Capacitance and Inductance)
For reactive elements connected in series, net reactance is the scalar sum of inductive reactance (positive in the mathematical complex plane) and capacitive reactance (negative in complex plane). Pure reactance values depend on the rates of change of current and voltage, whereas pure resistance values do not. Phenomena of this type are caused by differences in the timing of the peaks (phase angles) of the voltages or currents in the various reactive components. These reactive effects can be important in biventricular pacing threshold measurements (see later discussion). The component of reactance that is most relevant to both pacing electrodes and cell membranes is capacitance, with inductance being much less important. For example, the cardiac action potential spreading throughout the heart generates a changing magnetic field that transiently stores a very small amount of energy. However, the changing magnetic field generated by spread of the action potential is so small that it is not clinically significant except in the research setting.3
(remembering that and that the voltage across a capacitor at time t is
).
Cellular Aspects of Myocardial Stimulation
Cell Membrane Characteristics
Cell membrane characteristics are major determinants of tissue excitability. The membrane of the cardiac myocyte is composed principally of phospholipids, cholesterol, and proteins.4 The membrane phospholipids have a charged polar headgroup and two long hydrocarbon chains arranged as shown in Figure 1-1. The cell membrane comprises two layers of phospholipids with their hydrophobic aliphatic chains oriented toward the central portion of the bilayer membrane and their polar headgroup regions toward the outside boundaries of the membrane. Because the membrane is composed of two layers of phospholipids, the polar regions of the phospholipid molecules interface with the aqueous environments inside and outside the cell. The lipid-soluble hydrocarbon chains are forced away from the aqueous phase to form a nonpolar interior.
Determinants of the Resting Transmembrane Potential
Relatively large gradients of individual ion concentrations exist across the cardiac cell membrane.5 The gradient of sodium ions (Na+) across the membrane is approximately 145 millimoles per liter (mmol/L) outside to 10 mmol/L inside. In contrast, the potassium ion (K+) concentration outside the cell is approximately 4.5 mmol/L, whereas the inside concentration is 140 mmol/L. In the absence of a cell membrane, both Na+ and K+ would rapidly move in a direction determined by the concentration gradient. The diffusion force tending to move K+ out of and Na+ into the cell is proportional to the concentration gradients of those ions. The potential energy attributable to the diffusion force (PEd) tending to move K+ out of the cell is given by the following equation:
where z here is the valence (the number of positive or negative electrical charges) of the ion, F is the Faraday constant (96,500 coulombs/equivalent), and Vm is the transmembrane potential difference (transmembrane voltage, measured in millivolts). During equilibrium, the total of the potential energies from diffusion and electric forces is zero, and no net ionic movement occurs. Therefore, the sum of Equation 1-1 and Equation 1-2 may be set to zero. This yields the Nernst equation, which describes (in measurable electrical units) the potential that must exist for a single ionic species, here K+, to be in equilibrium across the membrane of a resting cardiac cell:
Using known values for extracellular K+, Vm(K+) = −90 mV. When Equation 1-3 is solved using Na+ concentrations, a Vm(Na+) of +50 mV is obtained. Therefore, it is the equilibrium potential for potassium ion (not sodium ion) that is the major factor responsible for the resting transmembrane potential. This suggests that the resting membrane is more permeable to K+ than to Na+.
To calculate the transmembrane potential when multiple ionic species exist in different concentrations across the membrane, the Goldman constant field equation (modified by Hodgkin and Katz6) is used:
where PK+, PNa+, and PCl− are the cell membrane permeabilities for the respective ions. At physiologic concentrations, this equation yields a transmembrane potential of −90 mV (the equilibrium potential for K+). Equation 1-4 describes how resting potentials vary as sodium and potassium ion concentrations are changed. Because there is a passive leak of charged ions through the membrane, the resting potential would not exist at the level of −90 mV unless it were actively maintained. This is accomplished by two active transport mechanisms that exchange Na+ ions for K+ and calcium (Ca2+) ions.
Ion Channels
Protein molecules embedded within the cell membrane have numerous functions, including those of being ion channels and signal transducers. The concept of ion channels was proposed in the 1950s by Hodgkin and Huxley.7 However, it was not until the introduction of the patch clamp technique by Neher and Sakmann in 1976 that the properties of these channels could be directly studied.8,9 There are two basic types of ion channels, distinguished by the factors that control opening and closing of the channel. Ion channels at muscle fiber end plates are chemically gated by specific transmitters. The opening of these channels is triggered by the binding of acetylcholine, and their closing is induced by its unbinding. In neuronal axons, conduction is mediated by faster, voltage-gated channels. These channels respond to differences in electric potential between the inside and outside of the cell, across the membrane. Voltage-gated channels for sodium, potassium, and calcium appear to operate in similar ways, sharing many of the same structural features. In addition, each type of channel can be subdivided into several subtypes with different conductance or gating properties (Fig. 1-2).
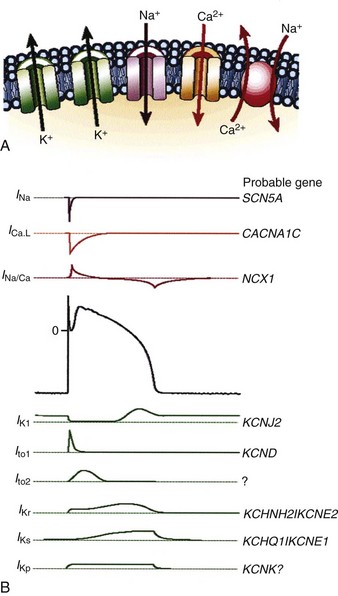
Figure 1-2 Ion channels underlie cardiac excitability.
(From Marban E: Cardiac channelopathies. Nature 415:213-218, 2002. ©Nature Publishing Group, http://www.nature.com.)
Voltage-Gated Channels
Voltage-gated channels open in response to an applied electric potential. The source of this voltage can be an action potential propagated from an adjacent cell or the electric field of an artificial pacemaker electrode. If depolarization of the membrane exceeds a threshold voltage, an action potential is triggered, resulting in a complex cascade of ionic currents flowing across the membrane into and out of the cell. As a result of this flow of charge across the membrane, the potential gradient across the membrane changes in a characteristic pattern of events that produce the cardiac action potential (Fig. 1-3).
Selective membrane-bound proteins (ion channels) determine the passive transmembrane flux of an individual ion species. The transmembrane currents determine or influence cellular polarization at rest, action potential depolarization and repolarization, conduction, excitation-contraction coupling, and myofibril contraction. The channels that regulate transmembrane conductance of Na+ and Ca2+ are voltage gated. The sodium channel is a large protein molecule composed of approximately 1830 amino acids.10 It contains four internally homologous repeating domains, believed to be arranged around a central water-filled pore lined with hydrophilic (“water-loving”) amino acids. It is estimated that there are 5 to 10 Na+ channels per square micrometer (µm2) of cell membrane. When an alteration changes the membrane potential to about −70 to −60 mV (the threshold potential), four to six positively charged amino acids move across the membrane in response to the change in electric field. This causes a change in the conformations of the channel proteins, resulting in opening of the channel. After a single Na+ channel changes to the open conformation, about 104 Na+ ions enter the cell. On depolarization of the membrane, the Na+ channels remain open for less than 1 millisecond (msec). After rapid depolarization of the membrane, the Na+ channel again changes to the closed conformation. In addition to the Na+ channel, specialized proteins are suspended in the cell membrane that have differential selectivity for K+, Ca2+, and Cl− ions, with much different time constants for activation and inactivation.
Sinoatrial and Atrioventricular Nodal Cells
In these nodal structures, depolarization is primarily mediated by inward Ca2+ conductance through specialized Ca2+ channels. There are two types of Ca2+ channels in the mammalian heart. The L-type channels are the major voltage-gated pathway for entry of Ca2+ into the myocyte, and they are heavily modulated by catecholamines.11 The T-type channels contribute to spontaneous depolarization of the cell associated with automaticity (pacemaker currents). The pore of the Ca2+ channel has a functional diameter of about 0.6 nanometers (nm), larger than that of the Na+ channels (0.3-0.5 nm).12 The selectivity for Ca2+ is high, up to 10,000-fold greater than that for Na+ or K+. The key elements are high-affinity binding sites for Ca2+, positioned along a single file pore. “Elution” of a Ca2+ ion occurs when another Ca2+ ion enters and is selectively bound.
Maintenance of Resting Membrane Potential
The resting membrane potential is maintained by the pumping of Na+ ions out of the cell and K+ ions into the cell. The Na+,K+—adenosine triphosphatase (ATPase) pump moves three Na+ ions out of the cell in exchange for two K+ ions moved into the cell.13–15 The basic unit of the Na+,K+-ATPase protein (pump) consists of one alpha (α-) and one beta (β-) subunit. The α-subunit is large (1016 amino acids) and spans the entire membrane, whereas the β-subunit is a smaller glycoprotein. There appear to be about 1000 pump sites/µm2 of cardiac cell membrane. The fully activated pump cycles about 50 to 70 times per second (interval of 15-20 msec/cycle). Similarly, the Na+-Ca2+ pump moves three Na+ ions out of the cell in exchange for one Ca2+ ion.16,17 Therefore, both transport mechanisms result in the net movement of one positive charge out of the cell, polarizing the membrane and maintaining a negatively charged interior. The function of both exchange mechanisms depends on the expenditure of energy in the form of high-energy phosphates and is susceptible to interruptions in aerobic cellular metabolism (e.g., during ischemia).
The Cardiac Action Potential
When the voltage gradient across the membrane of a myocyte decreases so that the inside of the cell becomes less negatively charged with respect to the outside of the cell, a critical transmembrane voltage difference is reached, the threshold voltage. At threshold, the cell membrane suddenly undergoes a further depolarization that is out of proportion to the intensity of the applied stimulus. This abrupt change in the potential across the membrane is the start of a cascade of inward and outward currents that together are known as an action potential.18
The cardiac action potential is an enormously complex event and consists of five phases19 (see Fig. 1-3): phase 0, the upstroke phase of rapid depolarization; phase 1, the overshoot phase of initial rapid repolarization; phase 2, the plateau phase; phase 3, the rapid repolarization phase; and phase 4, characterized by a slow, spontaneous depolarization of the membrane in cells with spontaneous pacemaker activity, until the threshold potential is again reached and a new action potential is generated.
Phase 1: Initial Repolarization
After voltage-dependent activation of the Na+ current in phase 0, the membrane potential rapidly changes from negative to positive. The increased conductance of Na+ is rapidly followed by voltage-dependent inactivation. Phase 1 is characterized by the transient outward K+ current (IKto). The outward movement of K+ is a major contributor to the various repolarization phases. It is complex and has a number of discrete pathways.20,21 Most K+ currents demonstrate rectification, that is, decreased K+ conductance with depolarization. The K+ currents include the instantaneous inward rectifier K+ current, the outward (delayed) rectifier K+ current, the transient outward currents, and ATP-, Na+-, and acetylcholine-regulated K+ currents. The initial repolarization, however, is mainly the result of activation of a transient outward K+ current and inactivation of the fast inward Na+ current.22 The transient outward K+ current has two components, one voltage gated and the other activated by a local rise in Ca2+.
Phase 2: Plateau
The net current during the plateau phase is apparently small, although the individual currents (inward Na+ and Ca2+ and outward K+) are each about an order of magnitude larger.23 Among the inward currents are the slowly activating Na+ current, a Ca2+ current, and an Na+-Ca2+ exchange current. Outward currents include a slowly activating K+ current (IKs), a Cl− current, a more rapidly activating K+ current (IKr), an ultra-rapidly activating K+ current (IKur), and the Na+-K+ electrogenic pump. During phase 2 of the action potential, the absolute refractory period, the cardiac cell cannot be excited by an electrical stimulus, regardless of its intensity.
Phase 4: Automaticity and the Conduction System
Automaticity is the property of certain cells by which they are able to initiate an action potential spontaneously. It has been known for centuries that the heart can exhibit spontaneous contraction even when completely denervated. Leonardo da Vinci observed that the heart could “move by itself.”24 William Harvey reported that pieces of the heart could “contract and relax” separately.25 Many cells within the specialized conduction system have the potential for automaticity.
Normally, depolarization is initiated at the SA node.26,27 Figure 1-4 shows action potentials from different types of cardiac cells.28 Rather than maintaining a stable resting membrane potential, the repolarization of the action potential is followed by a slow depolarization from about −71 to −54 mV, the threshold required to initiate another action potential. This slow, spontaneous depolarization drives cardiac automaticity and is related to a specialized current (If). In the case of AV nodal cells, the fast upstroke is carried predominantly by an inward Ca2+ current. Repolarization is caused by delayed activation of the K+ current. The balance of inward and outward currents determines the net “pacemaker” current and is finely regulated by both adrenergic and cholinergic neurotransmitters. In the presence of AV block or abnormal SA nodal function, AV junctional cells in the region of the proximal penetrating bundle usually assume the role of pacemaker at rates slower than that of the sinus node. In the absence of disease in the AV junction, the escape rhythm occurs with a frequency that is about 67% of the sinus rate.29
Artificial Electrical Stimulation of Cardiac Tissue
Artificial lipid membranes in their pure form are electrical insulators. The myocyte cell membrane (sarcolemma) is much more complicated. Specialized protein molecules in the membrane allow it to be conductive.30,31 These proteins, either singly or in certain groupings, form channels that open and close for transport of specific ions through the membrane in response to particular stimuli. The channel proteins are the end stages of processes that provide both active and passive transport of ions and molecules through the membrane.
The effect of the stimulus is to change the transmembrane voltage of nearby myocytes sufficiently so that depolarization begins in and spreads from these myocyte membranes. Propagation of the stimulus to nearby myocytes occurs because the local transmembrane depolarization changes the voltage gradient across adjacent membranes sufficiently to trigger depolarization of those membranes. To cause an action potential to spread throughout the whole heart, an electrical pulse must stimulate a minimum of approximately 50 closely coupled cardiac cells.5 The result is a self-regenerating action potential that progresses in a wavelike, relatively slow manner beyond the local effect of the pacemaker stimulus. Away from immediate vicinity of the electrode, transmission of depolarization and its velocity depend in part on the resistance and capacitance properties of the membrane, on the opening and closing of ion channels, and on ion flows through the sarcolemma.