Basic Principles of Radiation Therapy in Locally Advanced Carcinoma of the Lung
Bahman Emami
Nena Mirkovic
Julian Rosenman
Physical Aspects of Radiation Therapy
Ionizing radiation is the name given to high-energy particles or electromagnetic waves that are capable of stripping electrons away from their atoms, producing physical and biochemical changes when they interact with matter. Electromagnetic ionizing radiation is subclassified based on its source: gamma rays are emitted in discrete energy peaks by naturally occurring radioactive isotopes, while x-rays are broad-spectrum radiation produced by manufactured devices such as linear accelerators. Particle radiation beams used in radiation therapy include both types of electron, proton, and neutron beams.
When a beam of ionizing radiation interacts with matter, many electrons are ejected from their atoms. These high-speed electrons are then either reabsorbed, transferring their energy back into the material, or produce further ionization downstream along their pathways.
Units of Radiation
Radiation dose is defined as the energy absorbed per unit mass of the material. The radiation dose absorbed by living tissue is typically measured in grays (Gy), defined as 1 joule (J) of energy deposited per kilogram of mass. The older unit, the rad (radiation absorbed dose), was defined as 100 ergs/g and equals 0.01 Gy. One centigray (cGy) equals 1 rad, but modern usage calls for all radiation doses to be given in Gy. A roentgen (R) is a unit of radiation exposure in air and therefore cannot be used to describe deposited radiation dose. The activity of a radioactive isotope is measured in curies, which, by definition, is the number of disintegrations per second undergone by 1 g of radium.
Nomenclature
The International Commission of Radiation Units and Measurements has recommended definitions of important concepts in treatment planning in radiation therapy23:
The gross tumor volume (GTV) is defined as all known gross tumor that is seen on imaging, palpable, or determined in any other way. The gross tumor volume in lung cancer is determined primarily on lung windows of a computed tomography (CT) scan. There may be several millimeters of difference between the contours of GTVs determined on lung windows and those determined on mediastinal windows. In addition, there is considerable interobserver and intraobserver variability. Finally, there is now an entire literature on how to compensate for lung cancer motion in the treatment planning process; for example, see Rietzel33 or Boldea.6
The clinical target volume (CTV) is defined as areas suspected of harboring microscopic cancer cells. In lung cancer, a CTV generally includes microscopic extension of the primary tumor into the surrounding apparently normal tissue or clinically normal lymph nodes suspected of bearing microscopic disease. Several different CTVs can be defined, such as high-risk, low-risk, postchemotherapy, etc.
The planning target volume (PTV) is a margin of normal tissue surrounding the previously described volumes that is included in the radiation volume to account for organ and patient movement and inaccuracies of daily setup. Inaccuracies in daily setup can be minimized by using proper immobilization technology. Organ movement is much more difficult to deal with. In lung cancer, respiratory and cardiac movements can be considerable and need to be taken into account for planning (discussed subsequently).
Equipment Used in Clinical Radiation Therapy
High-energy x-rays produced by linear accelerators are by far the most commonly used beams for thoracic radiation therapy in the United States. Linear accelerators produce high-energy x-rays by bombarding a heavy-metal target (usually tungsten) with fast electrons. Low-energy x-rays, such as those produced by diagnostic x-ray units, have poor tissue penetration characteristics and deposit excessive doses in superficial tissues and bone,
making them unsuitable for the treatment of deep-seated thoracic tumors.
making them unsuitable for the treatment of deep-seated thoracic tumors.
Cobalt teletherapy units house the isotope cobalt-60 (60Co), which emits gamma rays. 60Co machines have been extensively used for thoracic radiation therapy in the past but have now been largely replaced by linear accelerators. 60Co beams have relatively low energy and poor edge definition; thus they deposit more dose in normal lung than do linear accelerator beams, likely increasing the risk of radiation pneumonitis. Thus the use of 60Co is no longer recommended for thoracic radiation therapy.
Biological Effects of Ionizing Radiation and Biological Basis of Radiation Therapy
It is often said that patients undergoing radiation therapy are having their tumors “burned out.” However, “burning” is not what is really happening. From the definition of a Gy (1 J/1,000 g) and the fact that 1 calorie (cal) = 4.184 J, it follows that it takes 4,814 Gy to deposit 1 cal of energy into 1 g of tissue. Since 1 cal is the amount of energy required to raise 1 g of water by 1°C, the amount of radiation needed to raise the temperature of the patient’s tissue by just 1°C is about 60 times a typical total therapeutic dose of 70 Gy. Thus cell death due to radiation therapy cannot be to the result of heating.
The critical target in radiation-induced cell death is in the nucleus and is probably DNA. Ionizing radiation damages DNA in at least four different ways: (a) double-helix strand breaks, (b) single-strand breaks, (c) base damage, and (d) damage to crosslinks, in which both DNA–DNA and DNA–protein cross-links are involved. Chromosomal damage is not detectable in interphase and appears only as the cells divide.
These physical and biochemical phenomena take place in fractions of a second at the time of the radiation exposure. Various nuclear and cytoplasmic molecular changes then follow, leading to loss of reproductive ability, cell cycle delays, somatic transformation, and mutations, among other effects.
Carcinogenesis
Ionizing radiation has the potential to induce cancer in any organ. The adult thyroid gland may tolerate as much as 40 Gy without significant changes, but in children who received between 2 to 8 Gy to the neck for thymic enlargement, the development many years later of thyroid carcinoma, usually of the papillary type, has been reported. The advent of three-dimensional radiation therapy and intensity-modulated radiation therapy (IMRT), which usually treat larger volumes of tissue (albeit at lower dose) than conventional radiation, raises the concern of radiation carcinogenesis (Fig. 114-1).21 In discussing the potential dangers of radiation carcinogenesis, it must be kept in mind that patients undergoing radiation therapy for non-small-cell lung cancer (NSCLC) have a far greater risk of dying of their current cancer than of one that might appear years later.
Cell Damage From Ionizing Radiation
When a cell is hit by an ionizing particle, three different kinds of damage may take place. Lethal damage occurs when the cell loses its capacity for unlimited proliferation. After radiation exposure, the cell and its subsequent progeny die, although—for most mammalian cells—death is delayed until that cell goes into mitosis. Potentially lethal damage consists of slightly less severe impairment of the cell’s proliferative ability, from which it may recover; after that, however, any modification in its environment is likely to interfere with its repair mechanism and cause the cell to die. Sublethal damage occurs when the injury induced by the ionizing radiation can be repaired by the cell. After exposure to ionizing radiation, such cells exhibit changes in their growth rate, including prolongation of the generation time and mitotic delay.
Factors Affecting the Biological Effects of Ionizing Radiation
Cell Sensitivity to Radiation
In clinical practice, a distinction must be made between cell sensitivity to radiation on the one hand and, on the other, tumor response and curability. Clinically, “radiation sensitivity” usually means rapidity of response—that is, how quickly the tumor shrinks after radiation has begun. For example, small-cell lung cancer often seems to disappear completely after only a modest dose of radiation (e.g., 30 Gy), whereas other tumors, such as non-small-cell lung cancer, may regress quite slowly. However, the rapidity of response to radiation cannot be used to determine the tumor control dose for that patient, as the rapidity with which cells express radiation damage is linked far more to their mitotic rate than to their inherent radiation sensitivity.
Oxygen Enhancement Effect (Reoxygenation)
The biological effect produced by a given dose of x-radiation is two to three times greater in the presence of oxygen than in its absence. This augmentation is called the oxygen enhancement ratio. Oxygen must be present during the radiation exposure. The oxygen enhancement effect is lessened or absent in high linear energy transfer (LET) radiations, such as alpha particles, fast neutrons, and pi mesons (see below). Although increasing concentrations of oxygen result in more sensitization to radiation, Gray19 reported that no significant gain is observed when the oxygen pressure is higher than 30 mm Hg. Suit and Shalek43 have suggested that the fraction of hypoxic cells in a tumor may negatively affect the tumor’s response to radiation and thus the probability of controlling it by radiation therapy. Tumor hypoxia may be reduced by the use of fractionated radiation, because reduction of the tumor size allows better oxygenation of the remaining cells.24 The observed lack of oxygen dependence for neutron therapy is one of the reasons for its continuing use.
Linear Energy Transfer
LET represents the energy transferred by an ionizing particle per unit length of pathway. The LET of an ionizing particle depends in a complex way on the energy and charge of the particle: the greater the charge and the lesser the velocity, the higher the LET.
The cytotoxic effect of radiation depends on the density of energy release (LET); therefore equal doses of various types of radiation can produce different biological effects on the patient’s
tissue. The term relative biological effect (RBE) was established to compare the biological effectiveness of a given ionizing radiation with a certain standard, represented by 250-kV x-rays. For instance, 60Co has a relative biological effect of about 0.95, and neutrons have a relative biological effect of between 2.0 and 2.5, depending on their energy.
tissue. The term relative biological effect (RBE) was established to compare the biological effectiveness of a given ionizing radiation with a certain standard, represented by 250-kV x-rays. For instance, 60Co has a relative biological effect of about 0.95, and neutrons have a relative biological effect of between 2.0 and 2.5, depending on their energy.
Repair of Radiation Damage (Dose Fractionation)
Radiation therapy is usually given in daily fractions, usually five times per week, on the presumption that normal cells generally have a greater and faster capacity to repair sublethal damage and undergo more cell repopulation between fractions than does tumor. This time–dose relation depends in a complex way on the individual sensitivity of the cells and their ability to repair themselves, size of the radiation fractions, total dose given, time between fractions and duration of overall treatment, initial hypoxic subpopulation, reoxygenation taking place throughout the fractionated therapy, and type of ionizing radiation used.
In radiation treatment planning, the specifications should include not only the volume of tissue treated and the total dose given but also the number of fractions and the overall period of time during which they are administered.
Radiosurgery, as opposed to standard fractionated radiation therapy, employs at most a few fractions of treatment or just one. The intent is not to exploit the biological difference between tumor and normal tissue but to use a very tight radiation dose distribution and kill most of the tissue within the tumor volume.
Dose Fractionation in Radiation Therapy of Lung Cancer
In the United States, typical dose fractionation used in lung cancer treatment is 1.8 to 2 Gy per fraction, delivered as one fraction per day, for an overall treatment duration of 6 to 7 weeks. In an effort to increase therapeutic efficacy, different fractionation schedules have been explored, generally aimed at increasing the number of daily fractions and decreasing the overall treatment duration. The stated rationales for such an approach are several. If the cells are most sensitive in G2/M phase of the mitotic cycle, increasing the number of fractions should theoretically increase the probability of strike in the most sensitive phase. Furthermore, as the number of surviving cancer cells is reduced by the initial part of therapy, the remaining cells proliferate more rapidly—a phenomenon known as accelerated repopulation. More frequent radiation treatments mean that the entire treatment time is decreased, as is the time tumor has to repopulate. Finally, by splitting the daily radiation dose into two
smaller fractions, the late (>6-month) side effects of radiation are thought to be reduced. However, despite the attractive biological concept, the improved efficacy of such regimens has not been confirmed in extensive clinical research involving NSCLC, such as the trial reported by Sause and associates.39 For small-cell lung cancer, the situation may be different, as reported by Turrisi and colleagues.44
smaller fractions, the late (>6-month) side effects of radiation are thought to be reduced. However, despite the attractive biological concept, the improved efficacy of such regimens has not been confirmed in extensive clinical research involving NSCLC, such as the trial reported by Sause and associates.39 For small-cell lung cancer, the situation may be different, as reported by Turrisi and colleagues.44
Process of Radiation Therapy
The process of radiation therapy begins with an evaluation and staging of the patient, both anatomically and pathologically. Comprehensive evaluation of the physiologic and functional status of the normal tissue organs—such as lungs, heart, and spinal cord—are an essential part of this evaluation. After the regimen to be used has been decided on (e.g., conventional fractionation, hyperfractionation, radiation plus chemotherapy), the technical process of administering radiation therapy begins with simulation. In the past, this planning process was done with a single radiograph, called a simulator film, which is a plane radio- graph with an overlaid grid for measurement purposes. On obtaining the simulation film, usually in an anteroposterior or lateral projection, a portal would be outlined by the radiation on- cologist that presumably contained the area of gross tumor as well as areas of subclinical disease and outlined the area to be radi- ated. After appropriate measurements of the dimensions of this area and the thickness of the patient, calculations would be carried out and the delivery of treatment would commence. This technique is currently labeled two-dimensional (2D) radiation therapy.
In the 1990s, the advent of computer technology led to development of three-dimensional conformal radiation therapy (3D-CRT), which represented a radical change in practice. Instead of doing the treatment planning on a plane film, one starts by obtaining a CT scan of the patient’s chest in the treatment position. An isocenter for the treatment is then selected and marked on the patient; usually it is at or near the center of the tumor volume. The patient is then excused and the process of treatment planning begins.
3D radiation treatment planning begins by identifying the gross tumor volume (GTV), clinical target volume (CTV), and planning target volume (PTV) as defined by the ICRU-50 recommendations discussed above. Delineation of these volumes takes a great deal of knowledge and skill and still remains significantly operator-dependent. For example, what window setting of the CT scan is most representative of the actual pathology? In general, the GTV will not be the same if it is determined on different CT window settings.
Delineation of the CTV can be even more problematic than that of the GTV. Recall that, by definition, the CTV represents the volume of tissue at risk for tumor but one in which no tumor can be imaged. For lung, this is the microscopic extension of the primary tumor into the surrounding apparently normal tissue or clinically normal lymph nodes suspected of bearing microscopic disease. Recently it has been the trend to make the CTV as small as possible, not treating the radiographically negative mediastinum. Advocates of this point of view stress that there is no evidence of a significant recurrence rate in the untreated mediastinum. They also point to the potential for esophageal morbidity in treating the entire mediastinum. The counterargument is that the source of tumor recurrence is always difficult to determine and that it is naive to think that just because a lymph node is not enlarged on CT means that it does not contain tumor, especially if the patient is known to have positive mediastinal nodes. In addition to the “small field/big field” argument, there is significant uncertainty as to whether to change the original CTV if tumor regression takes place after induction chemotherapy. At the University of North Carolina, we have taken the position that the CTV should not just be a mathematical expansion of the GTV but should include some of the proximal uninvolved mediastinum with the radiation fields limited by normal tissue tolerance (see below). We tend to regard tumor volumes that have regressed as a result of induction chemotherapy as still needing treatment, but to a lower radiation dose.
Particular care must be taken to deal with tumor motion due to breathing. Four general approaches have been proposed. The first and simplest is to observe the patient under fluoroscopy and draw an additional volume outside the CTV (the PTV) that is an envelope of the tumor movement. This approach will, of necessity, result in an increased volume of lung to be treated but often will still lead to acceptable treatment volumes. The second approach is to have the patient hold a deep breath during treatment (deep inspirational breath hold, or DIBH). (See, for example, Stock and colleagues.42) DIBH not only arrests tumor motion but also has the advantage of thinning the lung around the tumor, so that less normal lung is treated. Unfortunately, many lung cancer patients have COPD and cannot hold their breath for long. A third approach is known as gating. See, for example, Berman et al.4 Gating can be either active or passive—that is, with patient cooperation or not. The idea is to leave the accelerator on only during times when the lung is at a predetermined expansion. The treatment is, in effect, “strobed.” A fourth approach, more theoretical than currently practical, is to track the patient’s breathing, making adjustments in the accelerator treatment head or table movement to keep the tumor at isocenter. (See, for example, Zhang.49) Although this method requires the least cooperation on the part of the patient, it has proved technically difficult to implement and requires a continuous source of tumor position updating, which is not yet available on conventional linear accelerators.
The Use of Pet for Radiation Treatment Planning
A significant problem in defining GTV is distinguishing between the actual tumor and postobstructive atelectasis or pneumonia. Inclusion of other imaging modalities, such as positron emission tomography (PET), may further improve tumor delineation. PET scanning has shown significant promise in both initial staging of lung cancer, as noted by Caldwell8 and Haberkorn20 and their associates, and target volume delineation for 3D-CRT, as reported by Erdi and colleagues.14 Nodal involvement has been detected by PET scan in 80% to 100% of the studies in a meta-analysis comparing the efficacy of PET versus CT in the detection of nodal metastases reported by Dwamena and coworkers.12 In the Leuven Lung Cancer Group experience of the evaluation of 980 lymph node stations, the accuracy of PET was 85% (visually, 90%), as compared with 64% for the CT scan. The PET scan has been shown to be of significant help in distinguishing gross tumors from atelectasis or postobstructive phenomena. Fusion of PET images into treatment-planning CT scans is becoming an increasingly routine practice.29
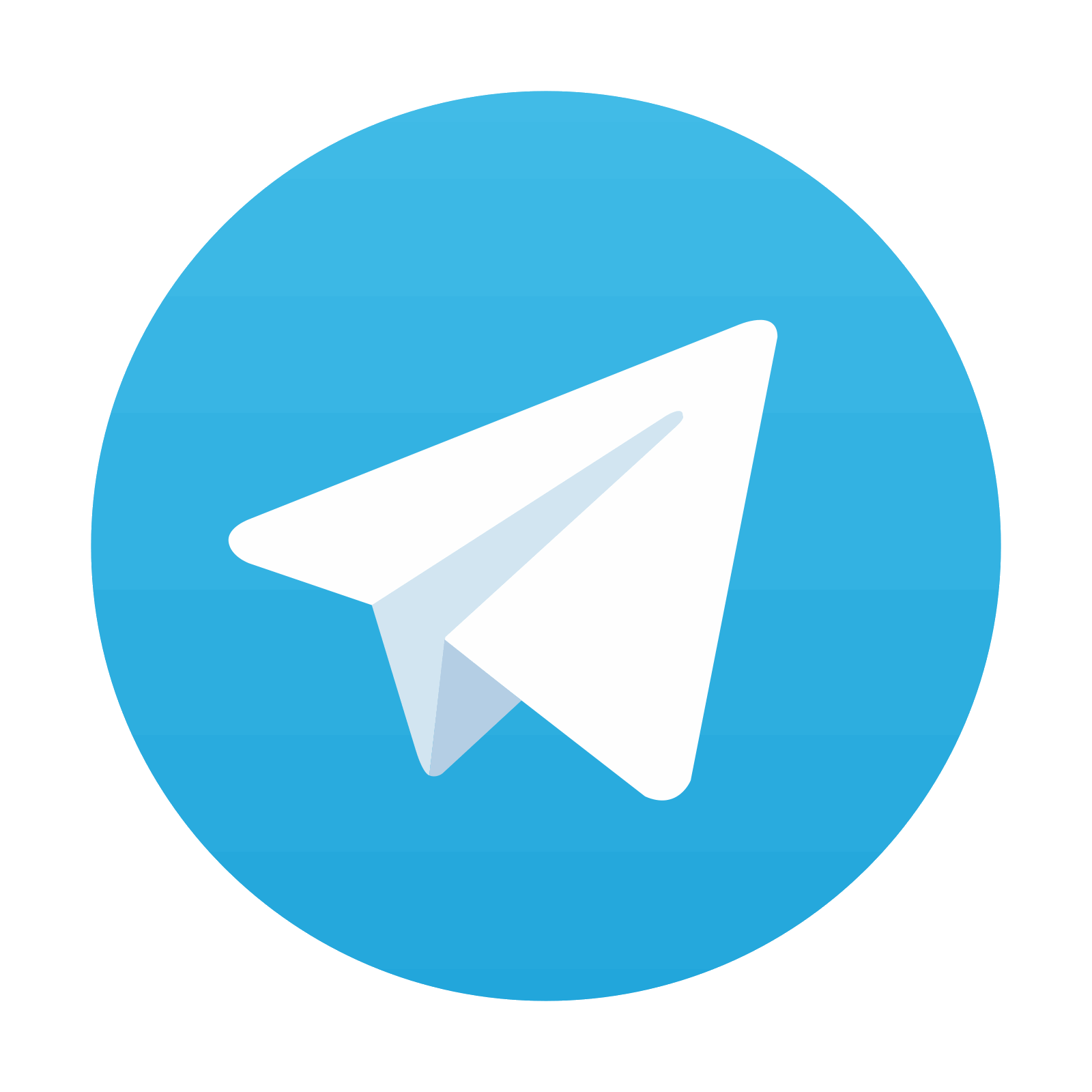
Stay updated, free articles. Join our Telegram channel
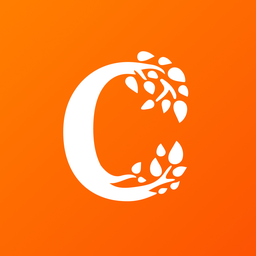
Full access? Get Clinical Tree
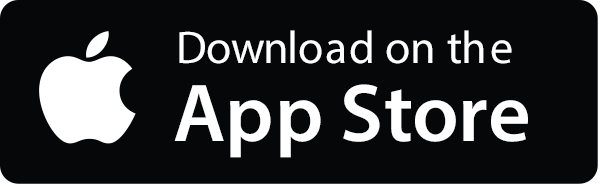
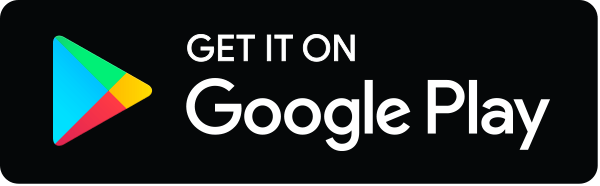