Echocardiography utilizes ultrasound to produce images of the heart and vascular structures (using M-mode, two-dimensional [2D], and and three-dimensionall [3D] echocardiography) and to provide information about the direction and velocity of blood flow within these structures (using spectral Doppler and color flow Doppler mapping). This chapter presents a basic review of the physics of ultrasound and the principles of cardiovascular ultrasonic imaging for all clinicians and medical personnel who perform echocardiography.
Ultrasound refers to sound waves with a frequency well beyond the range of human hearing (the normal human range is 20-20,000 Hz). Because the ability of ultrasound waves to penetrate human tissue carries an inverse relationship to the frequency of the transmitted ultrasound (as shown in Table 1-1), the range of frequencies that are suitable for transthoracic echocardiography generally falls between 2 to 10 MHz,1 which corresponds to an image depth of 6 cm in premature neonates (using a 10-MHz probe) down to 30 cm in adults (using a 2-MHz transducer). High ultrasonic frequencies are required to produce the necessary resolution for diagnostic imaging of small cardiovascular structures in newborns (Table 1-1). Because image resolution is directly related to the ultrasound frequency, the highest frequency that can penetrate to the structure of interest is generally preferred for M-mode, 2D, and 3D echocardiography. The opposite is true for Doppler and color flow mapping, in which lower ultrasonic frequencies generally provide better information.
Echocardiography utilizes properties of sound wave interaction with human tissue called reflection and scattering (Figure 1-1). The human body consists of various tissues, each with a different composition and density, that result in a sound-related property called acoustic impedance that is specific to the tissue. When sound propagates through a smooth and long interface between homogeneous tissues with two different acoustic impedances (such as between blood and endocardium), both transmission and reflection of the sound wave occur; the degree of transmission versus reflection depends upon the degree of matching between the two acoustic impedances (the greater the degree of mismatch, the greater the amount of reflection). Reflection of sounds waves is similar to reflection of light upon a mirror or a flat and smooth surface. Most human tissues, however, are either irregular or inhomogeneous. Reflection of sound in these irregular surfaces occurs in all directions, called “scattering,” similar to the reflection of light upon a ground glass or a wall with an irregular surface. Depending on the intensity of sound waves that reflects. or scatters, back to the transducer (we will call this “echo signals”), numerical values can be calculated and displayed as shades of gray on the display monitor. To obtain an echocardiographic image, ultrasound is created by a transducer, a device containing a material possessing piezoelectric properties (such as quartz or titanate ceramics) that enables the conversion from electrical to mechanical energy (and vice versa).2,3 When stimulated by electric current, these materials vibrate and produce an ultrasonic wave of predictable frequency. Therefore, through the use of brief electrical stimulation followed by immediate dampening of the transducer, a short pulse of ultrasonic energy can be emitted (each pulse is composed of several wavelengths of sound). Ultrasound pulses from the transducer travel through the tissue, and
when different tissue interfaces are encountered, various sound signals reflect or scatter (echo) back to the transducer. Because the speed that sound travels in human tissues is relatively constant (approximately 1,540 m/s),1 the distance to each tissue interface can then be calculated according to the time that the pulse of ultrasound travels to and from each tissue interface, using the formula
where
D = distance to tissue interface (such as between endocardium and the blood in the heart chamber, between subcutaneous tissue and pericardium, etc.)
T = time from the beginning of ultrasound pulse initiation to the return of the echo signal
C = speed of sound in human tissue (1,540 m/s)
Returning echo signals from the various tissue interfaces produces vibration of the piezoelectric material in the transducer, which in turn creates electric currents. The computer plots these currents as shades of gray to represent the intensity of the echo signals on the vertical axis according to the calculated distance between the transducer and each tissue interface.
M-mode, short for motion mode, is one of the oldest ultrasound techniques. It allows for assessment of motion of various structures of the heart along a single line. In a structurally normal heart, because the anatomy of the heart is known, each echo signal (gray dot) produced by the M-mode can be read as representing a certain interface between various structures (Figure 1-2). Because the heart is a moving organ, it is of interest to see the relative movement of these parts with time. For example, the relative movement of intraventricular septum to left ventricular (LV) posterior wall in systole compared to diastole provides information on LV systolic function (see Chapter 5). The M-mode images are created by repetitively sending and receiving ultrasound signals through the same path. To do so, the transducer will have to send an ultrasound signal, wait to listen for echo signals for a finite time, and then send another signal and listen once more. Because the speed of sound in the human tissue is relatively constant, there would be a specific amount of time, depending upon the depth of tissue, that an echo signal can be sent and received by the transducer before it can emit the next ultrasound pulse. The relationship between time (T) and depth (D) can be calculated using the formula given in the previous section, D =C × T2.
In reality, when an M-mode echocardiogram is performed, one does not choose the time between each pulse (T), or how often to send the ultrasound pulse in 1 second, also known as pulse repetition frequency (PRF). Instead, the machine has a user-defined depth adjustment that it uses to calculate the PRF. The image of M-mode echocardiography is made by putting the new “column” of gray dots to the right of the previous one (Figure 1-2). As such, the x-axis on an M-mode image represents time and the y-axis represents distance from the transducer, with the nearest distance on the top and the farthest distance on the bottom. It can also be seen that, for M-mode as well as other forms of echocardiographic imaging, the time that the machine sends and receives an ultrasound pulse has an inverse relationship to the depth of imaging: the deeper the depth, the longer it will take to obtain each line, and the lower the maximum PRF (Maximum PRF = 1/T). This has the potential to lower time (temporal) resolution. However, because the speed of sound is very fast relative to the depth of the human body, temporal resolution is excellent for M-mode echocardiography.1,3 This might not be the case in 2D or 3D echocardiography, especially when color flow mapping is also used, as will be discussed later in this chapter.
For M-mode echocardiography, a single unit of piezoelectric material is used to send and receive ultrasound to create an image which is essentially a one-dimensional plot of cardiac or vascular structures against time (Figure 1-2). It can give a good amount of information on the length of cardiac structures (such as wall thickness and chamber size) and how each structure moves in relation to other structures (such as the shortening fraction and ejection fraction). However, M-mode does not give any information on what each structure looks like unless one has a great imaginary capability (and most people do not).
With the advances in the computing technology, two-dimensional (2D) echocardiography (previously known as B-mode echocardiography) has now become the standard for cardiac imaging. For this form of echocardiography, images are created by sending and receiving multiple lines of ultrasound beams arranged in a fan-shape orientation, wide enough to visualize the area of interest. In the past, this was produced by automatically rotating the transducer back and forth (mechanical transducer), but currently it is performed by having an array of separate piezoelectric elements sending and receiving ultrasound signals at the same time or using a tiny fraction of time difference in the stimulation of the individual elements.2 In the transducer, piezoelectric materials are placed in a linear array such that successive ultrasound beams are directed with a very small change in angle (phased-array transducer). Each column of returning signals is then assembled with the previous column to create a “sector” scan (a collection of scan lines) that looks like a piece of pie (Figure 1-3). This image represents a 2D image of the heart along the scan plane at a particular point in time. When the transducer completes its round of sending and receiving echo signals throughout the sector, it begins the next cycle and the new image is created when the previous image disappears, in the same fashion as in a movie. Similar to a movie, when the images are created at a high enough frequency (frame rate), the motion appears continuous.
In 2D echocardiography, an operator can gain more complete information of the three-dimensional (3D) nature of a cardiac structure by moving (sweeping) the transducer and creating a mental picture of the 3D cardiac structure. Using this method, with adequate training, the diagnosis and understanding of 3D cardiac structure and function can be achieved with a high degree of accuracy. However, in certain circumstances, a true 3D rendering of a cardiac structure is desirable, such as when presenting the heart valve pathology to a cardiovascular surgeon in preparation for cardiac surgery. Similar to how a 2D image is obtained by putting together multiple lines of echo signals from a small linear array, a 3D echocardiogram is obtained by sending and receiving multiple scan lines from a 2D array containing a large number of elements arranged a tiny distance apart on a square or rectangular matrix. A pyramidal volume of gray-scale dots is then created for each time frame, resulting in a 3D rendered image. As with 2D imaging, the process is repeated continuously and rapidly to give the appearance of real-time motion (also known as four-dimensional, or 4D echocardiography). Because one cannot fully appreciate complex spatial relationships on a rendered 3D image displayed on a 2D computer monitor, it is often necessary to post-process the 3D dataset (using such maneuvers as image cropping and rotation) in order to develop a more complete 3D understanding of the structure of interest (such as a heart valve or a septal defect). Imaging with 3D echocardiography is beyond the scope of this book and will be omitted for simplicity.
Resolution of a 2D image is further divided into (1) detail resolution, which is the ability of the echocardiography to differentiate two adjacent points (or structures) as separate; (2) contrast resolution, which is the ability of the machine to differentiate between two tissue densities; and (3) temporal (time) resolution, or the ability to display a moving cardiac structure with a smooth transition between frames.
Detail resolution is further divided into resolution along the ultrasound beam, or axial resolution, and resolution between ultrasound beams, or lateral resolution (Figure 1-4). For most ultrasound images including echocardiography, axial resolution is better than lateral resolution.
FIGURE 1-4.
Axial resolution and lateral resolution. Axial resolution is the ability to distinguish between two structures in axial dimension and therefore measured parallel to the ultrasound beam. Lateral resolution is the ability to distinguish between two structures in lateral dimension and therefore measured perpendicular to the path of ultrasound beam.

Axial resolution of echocardiography is largely dependent on the frequency of the ultrasound used to obtain the image (Table 1-1). The higher the frequency, the shorter the wavelength of the sound wave, resulting in better axial resolution. This is because when the transducer sends out an ultrasound beam, it does so by creating a short train of sound waves (2-5 wavelengths) and then waits to listen for the echo signals. Hence, the shortest distance between two points that can reflect the sound back and be resolved by the transducer as different points is equal to the length of the ultrasound pulse divided by 2.2 If the pulse consists of two wavelengths, the resolution will be equal to the wavelength of ultrasound wave (Table 1-1). As a result, the shorter the wavelength (the higher the frequency), the shorter the pulse duration can be made, and the better the resolution. However, since the higher-frequency sound penetrates tissue less than lower-frequency sound, there is a trade-off between the ability to obtain better resolution 2D images and the decreased penetration from the higher frequency. Consequently, one should use the highest frequency that will penetrate to the structure of interest. In general, a transducer with sound frequencies of 2 to 4 MHz is used in adults, 4 to 6 MHz in children, and 6 to 12 MHz in neonates. The more premature the newborn, the higher the frequency of a transducer that can be used because of smaller chest size (and resultant smaller depth of penetration).
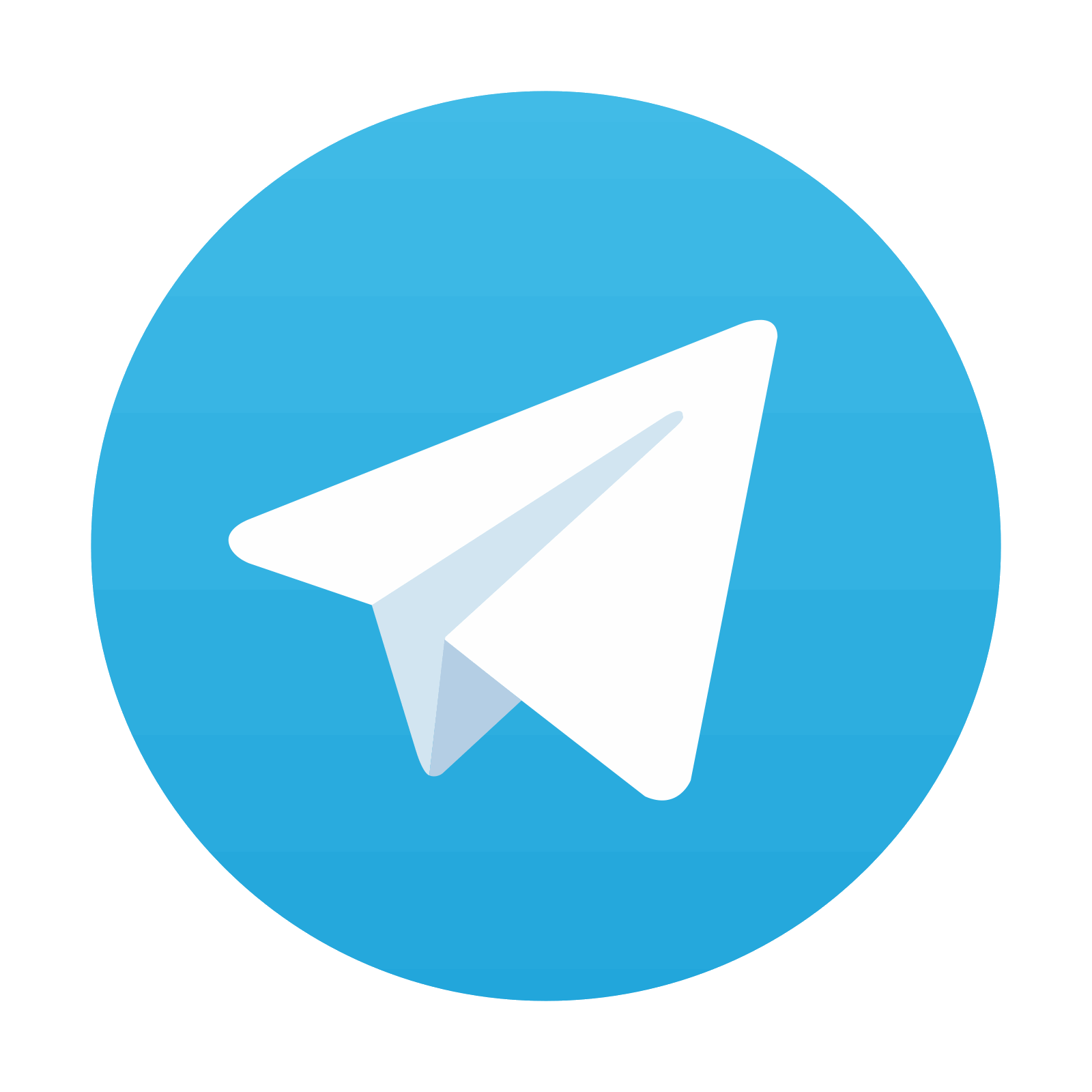
Stay updated, free articles. Join our Telegram channel
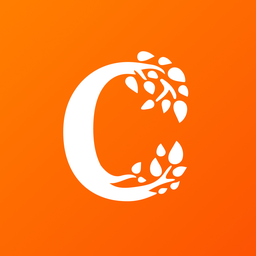
Full access? Get Clinical Tree
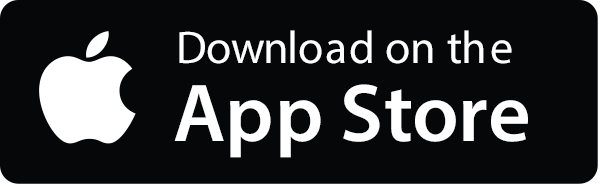
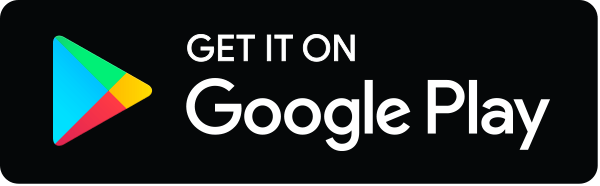