Fig. 3.1
Oxygen delivery (VO2ML) and carbon dioxide removal (VCO2ML) as a function of ECMO blood flow (BF). Operative range of BF of main CO2 removal techniques is represented. VV-LF-ECCO 2 R venovenous low-flow extracorporeal CO2 removal, VV-ECCO 2 R venovenous extracorporeal CO2 removal, VV ECMO venovenous ECMO, VA ECMO venoarterial ECMO
Oxygen and carbon dioxide pressure gradients between the sweep gas flow and the blood are the fundamental determinants of the gas transfer. In turn, these pressure gradients are dependent on metabolism and blood transport of oxygen and carbon dioxide.
3.3 Oxygen
Normal oxygen consumption for a healthy adult at rest is about 250 mL/min (5–8 mL/kg/min). Oxygen consumption may significantly increase during exercise, shivering and fever, but also, less noticeably, with increased level of catecholamine (restlessness, pain, exogenous therapeutic administration), increased work of breathing and increased thyroid hormones. Conversely oxygen consumption is reduced by hypothermia, sedation, paralysis and hypothyroidism [7].
Oxygen is used in mitochondria for substrate oxidation, which leads to production of energy and carbon dioxide. Therefore, the oxidative metabolism generates the partial pressure gradient that drives the oxygen from the outer ambient to the cell mitochondria. Respiratory and cardiovascular systems are adaptive mechanisms developed by multicellular organisms to guarantee the oxygen supply to any single cell of the body.
We will follow the path of oxygen from the ambient air to mitochondria to elucidate the main physiologic aspects of oxygenation [8].
Partial pressure of oxygen of inspired gases (pO2insp) is determined by the inspired oxygen concentration (FiO2) and the barometric pressure (pB):


The alveolar partial pressure of oxygen (pO2alv) is lower compared to pO2insp due to the added water vapor and the balance between oxygen removal by pulmonary capillary and oxygen replacement by alveolar ventilation.
Subsequently oxygen passes from the alveolar gas into the blood, mainly into the erythrocytes, by a passive diffusion process. In healthy lungs, diffusion is very efficient due to the extremely limited thickness of the alveolar-capillary barrier; therefore such equilibrium is easily reached.
The oxygen solubility in plasma is minimal (the coefficient of solubility is 0.003 mL/mmHg per 100 mL of blood); therefore with a normal arterial pO2 of 100 mmHg, the oxygen dissolved in plasma is only 0.3 mL/dL, corresponding to an oxygen delivery of 15 mL/min, assuming a cardiac output of 5 L/min. Without hemoglobin, a cardiac output of 80 L/min would have been necessary to provide an oxygen delivery of 250 mL/min!
Fortunately, evolution has provided hemoglobin, which raises blood oxygen content exponentially, binding 1.39 mL of oxygen per gram if fully saturated (SatO2).
Hence, total oxygen content can be calculated as


At a normal hemoglobin concentration, the arterial blood oxygen content is about 20 mL/dL.
Once the pulmonary capillary blood is loaded with oxygen, the cardiac output (CO) is regulated to maintain the systemic oxygen delivery (DO2) at four to five times the consumption. Oxygen delivery is the arterial oxygen content times cardiac output, which is the oxygen delivered to the tissues each minute. DO2 depends on cardiac output, hemoglobin concentration, hemoglobin saturation, and dissolved oxygen.
In the peripheral arterial capillary, hemoglobin releases oxygen. Following a pressure gradient, it diffuses through the endothelium, the intracellular space, and the cellular membrane, reaching its final destination, the mitochondria. In the mitochondria pO2 ranges between 3.8 and 22.5 mmHg, but it varies between tissue, cells, and even regions of the same cell.
After transferring oxygen to the tissues, the capillary blood flows into the venous district where the oxygen content (CvO2) may be computed as
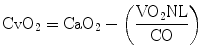
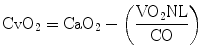
The right heart then drives the venous blood into the pulmonary circulation. There, venous blood is loaded of an amount of oxygen corresponding to that consumed by the tissues.
From the pathophysiologic point of view, the most important cause of acute hypoxic respiratory failure is maldistribution of ventilation (VA) and perfusion (Q). Following the three compartment lung model developed by Riley [9], the lung can be imagined as divided in three functional units characterized by different VA/Q ratios:
1.
Ideal lung, without alteration of the natural coupling between ventilation and perfusion, having VA/Q ~ 1
2.
Dead space, ventilated but not perfused alveoli, having VA/Q = ∞
3.
Intrapulmonary shunt, perfused but not ventilated alveoli, having VA/Q = 0
In this model, gas exchange can happen only in the ideal alveoli. Dead space has important effects on carbon dioxide elimination (see later). Conversely, as an effect of shunt, the blood flowing through a pulmonary parenchyma with VA/Q = 0 does not participate in the gas exchanges and is not oxygenated. Hence, part of the venous blood mixes with arterial oxygen content and determines hypoxemia. The shunt is usually characterized as a ratio (Qs/Qt) between the shunted blood (Qs) and the total pulmonary perfusion (Qt).
When the Qs/Qt is higher than 0.4, even providing inspiratory fraction of oxygen up to 100 % is not sufficient to ensure an adequate oxygenation, and some degrees of hypoxia should be expected. If Qs/Qt is higher than 0.4, oxygenation provided by the native lung cannot sustain vital oxygen delivery. In these extreme clinical conditions, ECMO may prove to be the only clinical solution (Fig. 3.2).
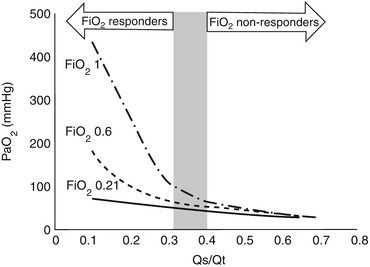
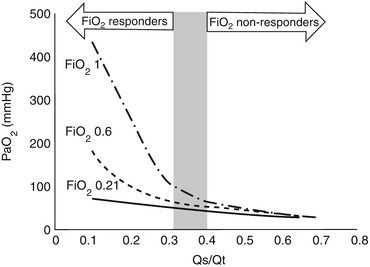
Fig. 3.2
Partial pressure of oxygen in the arterial blood (PaO2) as a function of pulmonary shunt (Qs/Qt), at various fractions of oxygen at the ventilator. If pulmonary shunt is higher than 0.4, vital arterial oxygenation may not be achieved even by 100 % oxygen supplementation at the ventilator
3.3.1 Oxygenation During VV ECMO
When the natural lung is ineffective in oxygenating the blood, a VV ECMO support may be employed.
The global physiology of oxygenation during VV ECMO may be elucidated by following the changes in blood O2 content along the circulatory system. Figure 3.3 is a schematic representation of a patient connected to a VV ECMO (panel a) and blood oxygen contents along the circulatory system (panel b).
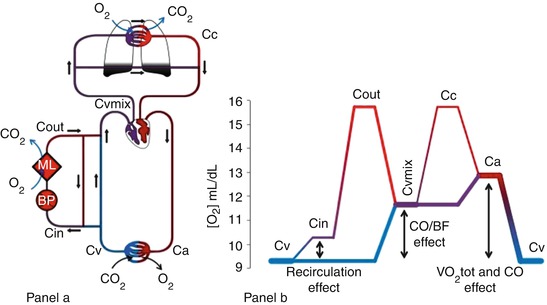
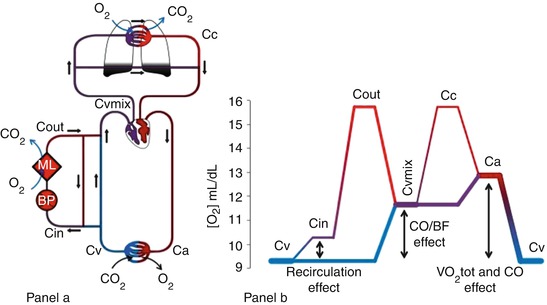
Fig. 3.3
Oxygen delivery and consumption during VV ECMO. Panel (a) The main determinants of blood oxygen content are represented. Oxygen content in the various blood compartments of the patient and ECMO circuit: Ca arterial, Cv venous, Cin circuit inlet, Cout circuit outlet, Cvmix mixed venous, Cc ideal unshunted pulmonary capillary. BP blood pump, ML membrane lung. Panel (b) Diagram representing the blood oxygen content of a patient (Hb 10 g/dL) connected to a VV ECMO support. The different sections of the venous, arterial, and extracorporeal circulation are distinguished by color and thickness. Blue lines represent deoxygenated blood, while red well-oxygenated blood. Thicker lines correspond to higher blood flows. Arrows represent the effects of recirculation ratio, cardiac output, and oxygen consumption on oxygen delivery during VV ECMO
Blood leaves the peripheral tissue with low oxygen content (CvO2). The blood pump generates the extracorporeal blood flow (BF) diverging part of the venous return towards the ML. In this process, part of the already-bypassed flow is drained back to the extracorporeal circuit. This results in a recirculating blood flow (R), which has detrimental effects on the oxygenation efficiency of VV ECMO (Fig. 3.4). Moreover, the R accounts for the difference in oxygen content between CvO2 and the oxygen content of the blood entering the ML (CinO2).
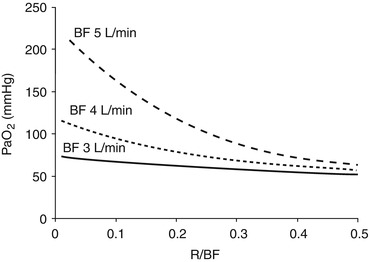
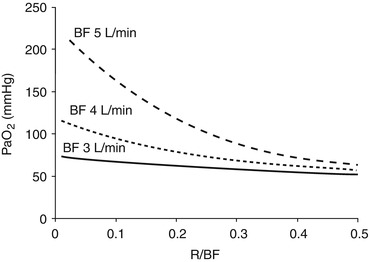
Fig. 3.4
Partial pressure of oxygen in arterial blood (PaO2) as a function of the fraction of the recirculating blood flow (R/BF) at different blood flows (BF). High recirculation fractions have extreme detrimental effects on the membrane lung oxygen delivery
Then, the ML loads the extracorporeal BF with oxygen (VO2ML) and raises the oxygen content in the outlet blood (CoutO2), as follows:


Subsequently, during VV ECMO, the blood returning to the right heart (the mixed venous blood, with oxygen content CvmixO2) is an admixture of the deoxygenated venous return and the well-oxygenated extracorporeal blood. The important role of the interaction between CO, BF, and R in determining the respective influence of these two components will be described later.
The resulting effect of VV ECMO application is the increase of the oxygen content of the blood returning to the lung. Fundamentally, VV ECMO improves arterial oxygenation increasing oxygen content of mixed venous blood.
CvO2 is eventually increased to CaO2 by the residual oxygenating capacity of the NL. The oxygen added to the blood by the natural lungs (VO2NL) is calculated as


The sum of VO2NL and VO2ML is equal to the total oxygen consumption of the patient:


Since most of the oxygen is transported bound to hemoglobin, the oxygen content of blood is highly dependent on the concentration and saturation of hemoglobin. An effective strategy in increasing DO2, after optimizing the ML and NL function, is increasing the hemoglobin concentration [10].
We will now address the roles of VO2ML, SvmixO2, and VO2NL in determining arterial oxygenation during VV ECMO.
3.3.1.1 VO2ML
The capacity of the ML of transferring oxygen is mainly determined by three factors:
1.
The intrinsic properties of the ML affect the oxygen passive diffusion from the sweep gases into the blood.
2.
The partial pressure gradient of oxygen between blood and sweep gases. The oxygen partial pressure in the sweep gases is determined by the FiO2. The oxygen transfer through the ML is affected by the ventilation/perfusion matching, the hemoglobin concentration, and the transit time. When the oxygen partial pressure increases in the blood crossing the ML, hemoglobin becomes fully saturated, and little additional oxygen, the physically dissolved, can be further loaded; therefore also an additional increase in GF will determine a minimal increase in the ML oxygen delivery. On the blood side, PinO2 and the resulting SinO2 highly affect the VO2ML. Recirculation, increasing PinO2, may vastly reduce VO2ML (Fig. 3.4).
3.
The extracorporeal blood flow is the major determinant of VCO2ML. Indeed, increasing the extracorporeal BF determines linear augmentation of VO2ML.
3.3.1.2 SvmixO2
During VV ECMO, SvmixO2 is mainly determined by the oxygen saturation of the blood leaving the tissues (SvO2), the ratio between BF and CO, and the presence of recirculation (Fig. 3.5).
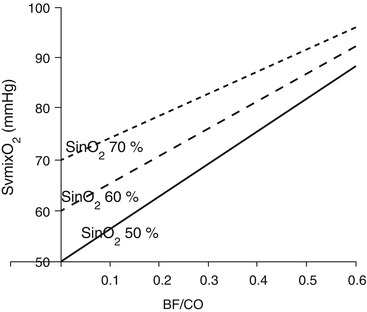
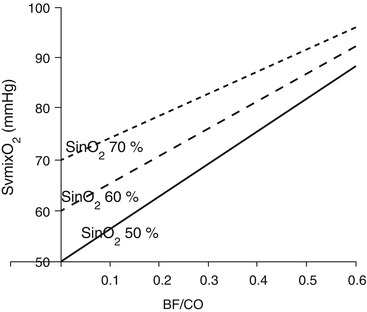
Fig. 3.5
Mixed venous oxygen saturation (SvmixO2) as a function of blood flow/cardiac output ratio (BF/CO), at different oxygen saturation in the blood entering the ML (SinO2)
As previously said, an increase in BF at constant CO and R always determines an improvement in arterial oxygenation and tissue oxygen delivery.
More complicated are the effects of CO changes on oxygen delivery and arterial oxygenation. Assuming stable BF and R, patients with elevated CO necessitate higher BF to achieve normal arterial PaO2 levels (Fig. 3.6, panel a). This does not necessarily means that a lower cardiac output is desirable. Indeed, DO2 depends on CaO2 and tissue perfusion. During arterial hypoxemia, more than ever, an adequate CO is essential to assure adequate oxygen delivery. Thus, increases in CO are usually associated with an increase oxygen delivery by the natural lung, assumed that the Qs/Qt is not changing. In this situation, a higher cardiac output provides higherVO2NL, CvO2, and consequently PvO2 (Fig. 3.6, panel b).
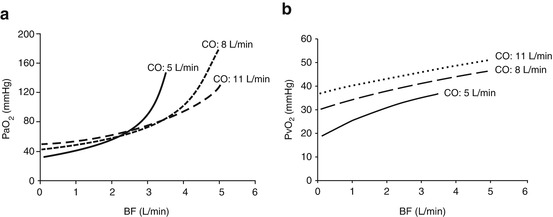
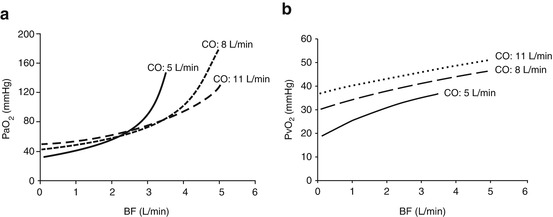
Fig. 3.6
The interaction between cardiac output (CO) and blood flow (BF). Panel (a) Partial pressure of oxygen in the arterial blood (PaO2) as a function of blood flow (BF), at different cardiac output (CO). Panel (b) Partial pressure of oxygen in the venous blood (PvO2) as a function of blood flow (BF), at different cardiac output (CO)
However, a rise in CO is usually a sign of augmented tissue oxygen requirements (e.g., fever, agitation, sepsis) and may change Qs/Qt and the R flow. During VV ECMO support, sudden changes of hemodynamic status are accompanied by complete alteration of the oxygenation steady state. Indeed, clinical experience teaches that in these scenarios CvmixO2 and CaO2 may unpredictably improve or worsen. Considering that many tissues have various oxygen requirements and vasculature, it is particularly difficult to predict the effect of a change in CO on oxygenation of different peripheral organs. Still, on this topic, scientific evidence is poor, and more research trials are needed [11] to better understand the complex pathophysiology of the effects of CO modulation on oxygen delivery.
3.3.1.3 VO2NL
The mixed venous blood is oxygenated according to gas exchange capability of the NL, which depends on the severity of the lung disease (mainly the intrapulmonary shunt fraction) (Fig. 3.7) and the ventilator setup (Fig. 3.8).
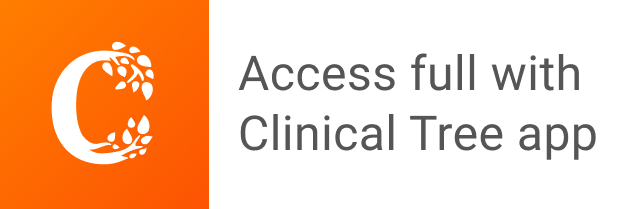