Barotrauma and Inhalation Injuries
Joseph B. Zwischenberger
Ryan Fischer
The term barotrauma is used to indicate damage induced by pressure applied to the airways and alveoli. Similarly, volutrauma refers to damage induced by excessive air volume in the airways and alveoli, which causes alveolar overdistention and stretching. Inhalation injury usually occurs during exposure to a fire, as the culmination of upper airway thermal damage, from inhaled toxins and gases, and because of systemic manifestations of injury. Ventilator-induced lung injury (VILI) is the cascade of pathophysiology induced by positive-pressure ventilation, which can incite or aggravate lung inflammation. Each of these circumstances yields parenchymal injuries that cause decreased gas-exchange efficacy and pulmonary compliance. This chapter outlines the pathophysiology, time line of progression, and treatment strategies of such conditions.
Barotrauma
Barotrauma may indicate damage at the cellular level, such as the parenchymal injury seen in adult respiratory distress syndrome (ARDS), or it may indicate extra-alveolar air caused by airway disruption, such as that seen with pneumothorax or subcutaneous emphysema. Although the etiology of the airway injury is pressure, the pathophysiology manifests itself in distinct clinical presentations.
Extra-alveolar Air–Associated Barotrauma Etiology and Management
Common manifestations of extra-alveolar air include pneumothoraces and subcutaneous emphysema. Subcutaneous emphysema usually is not clinically significant itself but rather an indicator of airway injury that may have other manifestations and requires prompt evaluation. Pneumothoraces can cause significant impairment of ventilation, gas exchange, and cardiac function, requiring prompt diagnosis and treatment. Macklin53 and Macklin and Macklin54 reported, more than 60 years ago, that any sudden increase in alveolar pressure may result in a gradient sufficient to disrupt the alveolar wall. Once ventilator-applied pressure ruptures the alveoli, air dissects along the loose connective tissues of the bronchovascular bundle or the interlobular septa medially toward the hilum and peripherally toward the visceral pleura. Because the mediastinal pleura is relatively weak and thin compared with the visceral pleura, air often dissects toward the hilum, where it can enter the mediastinum and form a pneumomediastinum. With subsequent rupture of the mediastinal pleura, air enters the pleural space, causing a pneumothorax. A continuum of fascial planes connects the soft tissue compartments of the mediastinum, neck, and retroperitoneum, also allowing decompression of the pneumothorax into these compartments, with subsequent formation of dissecting air pockets. Parker and coworkers68 reported that pressure- and volume-related alveolar rupture is the most common cause of pneumothorax in ventilated patients.
Several factors associated with barotrauma and volutrauma increase the risk for pneumothorax development. Amato and coworkers6 showed that high tidal volumes (≥12 mL/kg) and high plateau pressures produced a 42% incidence of pneumo- thorax and 71% mortality rate, as compared with incidences of 7% pneumothorax and 38% mortality rate with limited plateau pressure and tidal volume.43 Gammon and colleagues30 found that high positive end-expiratory pressure (PEEP) values and high minute ventilation also increase the risk of pneumothorax.
Unfortunately, because direct measurement of lung volume is difficult in ventilated patients, human studies have not distinguished the damaging effects of excessive airway pressure versus inspired volume as the cause of alveolar hyperinflation and injury. Colebatch and Ng16 retrospectively examined 14 men who developed pneumothoraces during shallow-water diving and compared their pulmonary mechanics with those of 34 healthy nonsmokers and 10 age-matched healthy male divers. Those who had developed pneumothoraces had stiffer airways and smaller airspaces, which increase the likelihood of barotrauma and interstitial gas. These mechanics may be analogous to those of patients with ARDS, who have markedly decreased compliance and smaller, thickened alveoli, posing an increased risk for pneumothoraces.
Placement of a tube thoracostomy (open or percutaneous) is often required for drainage of a pneumothorax, owing to the presence or potential of a tension pneumothorax or cardiac tamponade. Mechanically ventilated patients who develop a pneumothorax require tube thoracostomy because of the higher risk of tension pneumothorax. Pneumothoraces with a tension component impair cardiac venous return and ventricular filling. Drainage can be accomplished with urgent needle decompression and/or traditional chest tubes or image-guided catheter techniques, as reviewed by Woodside and colleagues.89 Placement and management of these tube thoracostomies are described in detail in Chapter 58. Although high airway pressures theoretically increase the magnitude of the air leak, evidence
from trials is sparse. Cerfolio and coworkers10 found that water seal was more effective than suction at stopping air leaks, although they also noted that large leaks probably would not respond to water seal.11
from trials is sparse. Cerfolio and coworkers10 found that water seal was more effective than suction at stopping air leaks, although they also noted that large leaks probably would not respond to water seal.11
Parenchymal Barotrauma and Volutrauma
VILI was first recognized as a distinct type of barotrauma by Webb and Tierney.88 Rats ventilated with high airway pressure (45 cm H2O) developed alveolar edema, hypoxemia (measured by the pressure/flow [P/F] ratio76), and decreased lung compliance; they usually died within 1 hour. This suggested that alveolar edema was caused by the depletion or inactivation of surfactant. Three of the main symptoms in ARDS are decreased lung compliance, alveolar edema, and hypoxemia,82 all of which presented in the rats. Later, Peevy and coworkers69 suggested that peak airway pressure was the most important mechanism of injury. In an isolated perfused rabbit lung model, they ventilated four sets of lungs with either low or high rates of gas flow while holding the peak inspiratory pressure (PIP) either low (27 cm H2O) or high (50 cm H2O). The high-PIP group had significant microvascular injuries. Using geometric and mathematical modeling to evaluate mean airway pressure, Marini and Ravenscraft56 found that, under conditions of passive inflation, mean arterial pressure correlated with arterial oxygenation, hemodynamic performance, and barotrauma.
Elevated PIP, high respiratory rates, oxygen toxicity, and alveolar overdistention combined account for ventilator-associated barotrauma. Likewise, Dreyfuss and colleagues26,27 found that intermittent positive-pressure hyperventilation with high inflation pressures produced pulmonary microvascular injury and capillary permeability edema in a rodent model. Large-animal models used by Tsuno and coworkers86,87 demonstrated that regional pressure–related overdistention results in a pattern of diffuse alveolar damage that is histologically similar to clinical ARDS, with similar pulmonary mechanics. In a sheep model of ARDS induced by moderately high airway pressures, Tsuno and colleagues86 described progressive deterioration in total static lung compliance, functional residual capacity, and arterial blood gases, with radiologic abnormalities emerging over time. At necropsy, there was severe pulmonary atelectasis, increased wet lung weight, and increased minimum surface tension of saline lung lavage fluid. In a later study,87 Tsuno and coworkers analyzed the histopathologic changes that occurred in the lungs of baby pigs after mechanical ventilation at a PIP of 40 cm H2O for approximately 1 day. They described a number of changes consistent with early ARDS, including alveolar hemorrhage, alveolar neutrophil and lymphocyte infiltration, alveolar macrophage and type II pneumocyte proliferation, interstitial congestion and thickening, emphysematous changes, and hyaline membrane formation. Further mechanical ventilation led to the additional finding of exudative organization similar to that seen in late ARDS. Control animals ventilated at 18 cm H2O did not manifest any significant histological findings.
Ultimately, overdistention rather than pressure probably accounts for the majority of ARDS-like changes. Using a rabbit model, Hernandez and associates35 found that chest wall restriction had the effect of limiting high airway pressure–induced lung injury in immature rabbits. Specifically, they found that inspiratory volume limitation with a full-body plaster cast
abrogated capillary permeability increases by PIP, suggesting that the volume distention, rather than the high PIP, produces the capillary damage. Dreyfuss and coworkers27 also demonstrated that mechanically ventilated rats at high pressure and low volume did not demonstrate capillary permeability changes, whereas those at low pressure and high volume had significant pulmonary edema and permeability, suggesting the predominant role of volume in the etiology of injury.
abrogated capillary permeability increases by PIP, suggesting that the volume distention, rather than the high PIP, produces the capillary damage. Dreyfuss and coworkers27 also demonstrated that mechanically ventilated rats at high pressure and low volume did not demonstrate capillary permeability changes, whereas those at low pressure and high volume had significant pulmonary edema and permeability, suggesting the predominant role of volume in the etiology of injury.
The Acute Respiratory Distress Syndrome Network Trial5 confirmed this finding in humans. Furthermore, because ARDS is a nonuniform disease, as noted by Gattinoni and associates,32 injury may be aggravated because preferential ventilation of normal alveoli leads to overdistention as the alveoli try to accommodate the increased tidal volume. This overdistention, in turn, may lead to further stretch injury.
Interestingly, the lung injury described by Dreyfuss and coworkers27 was markedly reduced by the application of 10 cm H2O of PEEP, suggesting that the unequal forces generated by repeated recruitment and derecruitment of lung alveolar units may be partially responsible for parenchymal damage (Fig. 74-1). This subset of volutrauma is sometimes termed atelectotrauma31 (Fig. 74-2).
PEEP acts as a pneumatic stent, preventing alveolar collapse at end expiration and minimizing shear forces associated with repeated opening and closing of collapsed alveolar units, as reported by Hudson.41 More recently, de Prost and colleagues21 showed that PEEP can reduce edema and the permeability of albumin into the alveoli, perhaps leading to decreased sepsis and inflammation. Malhotra55 also advocates the implementation of low tidal volume ventilation in ARDS patients.55 PEEP is not, however, always appropriate. Ranieri and associates73 examined the effects of PEEP on ARDS patients. Observing the pressure–volume loops on zero end-expiratory pressure (ZEEP) and PEEP, they found two distinct populations of patients. The first group demonstrated evidence of alveolar recruitment, with upward concavity of the pressure–volume curve during ventilation with ZEEP, which increased with PEEP. The second group demonstrated an upward convexity that was further aggravated with PEEP, suggesting volume displacement without alveolar recruitment and increased susceptibility to barotrauma.
PEEP may actually have no effect. In the National Heart, Lung, and Blood
Institute ARDS Clinical Trials Network, patients receiving a low tidal volume (6 mL/kg) and end-inspiratory plateau-pressure limit of 30 cm H2O had very similar outcomes with or without PEEP.65 Critics note the small difference between the high- and low-PEEP groups and that no static pressure curve was used to determine the lower inflection point in the high-PEEP group.
Institute ARDS Clinical Trials Network, patients receiving a low tidal volume (6 mL/kg) and end-inspiratory plateau-pressure limit of 30 cm H2O had very similar outcomes with or without PEEP.65 Critics note the small difference between the high- and low-PEEP groups and that no static pressure curve was used to determine the lower inflection point in the high-PEEP group.
The mechanism of barotrauma and volutrauma is a subject of intense investigation. Kawano and colleagues46 demonstrated immune involvement with a neutropenic rabbit model. They applied a saline lung lavage followed by controlled mechanical ventilation with tidal volumes of 12 mL/kg to three groups of rabbits. The normopenic controls developed high-permeability pulmonary edema with hyaline membrane formation. The second group was rendered neutropenic with nitrogen mustard pretreatment and lacked these pulmonary changes. The third group was also rendered neutropenic but was retransfused with granulocytes before pulmonary lavage and had similar findings to the normopenic group. In humans, Ranieri and coworkers74,75 demonstrated that concentrations of plasma and bronchoalveolar cytokines were significantly lower in patients receiving a protective lung strategy as compared with conventional mechanical ventilation. In addition, the Acute Respiratory Distress Syndrome Network trial3 demonstrated lower plasma levels of interleukin-6 in patients treated with protective ventilation. Current studies suggest that the physiologic changes seen with barotrauma and volutrauma involve both a mechanical and immune etiology for the development of lung injury. Recent recommendations from the National Heart, Lung, and Blood Institute Working Group on Acute Lung Injury and ARDS, which were summarized by Matthay and associates,57 suggest that an improved understanding of the genetics of individual responses is required for a more comprehensive understanding of such a multifactorial disease process.
Prevention and Management
Prevention and management strategies for volutrauma are similar. Ventilator strategies should target reducing airway pressures and volumes to avoid exacerbating volutrauma and thereby reducing endogenous inflammation. In a meta-analysis, Bidani and colleagues8 concluded that permissive hypercapnia allows avoidance of overdistention through pressure or volume limitation and may improve outcome. Laffey and others also considered permissive hypercapnia relatively benign.50 Slutsky,81 writing for the American College of Chest Physicians Consensus Conference, recommended that airway pressures should be limited to reduce tidal volumes under conditions of lung overdistention, while accepting the increase in arterial PCO2 levels (permissive hypercapnia). Hickling and associates39 suggested that lower tidal volumes of 5 to 8 mL/kg would prevent excessive alveolar distention.
Amato and coworkers6 directly compared mechanical ventilation with 6 to 12 mL/kg of tidal volume. Seven percent of the patients in the former group had clinical evidence of barotrauma, compared with 42% in the latter group (with mortality rates of 38% and 71%, respectively). More recently, the Acute Respiratory Distress Syndrome Network3 released their multicenter, randomized, controlled trial comparing 6 and 12 mL/kg of tidal volume, with plateau pressures below 30 and 50 cm H2O, respectively. The trial was terminated after 861 patients because the outcomes in the low tidal volume group were clearly superior. Specifically, mortality was 31% in the low tidal volume group and 40% in the higher tidal volume group (p = 0.007).
Other techniques, such as inverse-ratio ventilation, high-frequency jet ventilation, and extracorporeal life support (ECLS), along with pharmacologic manipulation with agents such as activated protein C, show promise for treatment of severe established ARDS and systemic inflammatory response syndrome rather than the prevention and treatment of volutrauma and are described in more detail in Chapter 75.
Inhalation Injuries
The most common pulmonary injury associated with burns is smoke inhalation. In fact, Ryan and coworkers77 described inhalation injury, along with advanced age (>60 years) and greater than 40% total body surface area (TBSA) burn, as one of the three most important risk factors for the determination of mortality following burn. Herndon and colleagues37 noted a 33% incidence of inhalation injury with major burn. Furthermore, Demling22 stated that these injuries account for 50% of burn deaths at the burn scene. Therefore an understanding of this malady is of particular importance for improved patient outcome.
Pathophysiology
Inhalation injury rarely implies a direct pulmonary parenchymal burn and is distinct from pulmonary edema resulting from overresuscitation. The upper airways absorb the majority of the heat, often resulting in swelling and possible obstruction of the upper airway. The lower airway and parenchyma usually receive adequately cooled air or gas due to the upper airway’s efficiency at cooling inspired volumes of gas.85 The usual etiology of lower airway injury is inhalation of smoke or chemical irritants released during the fire, and such injury may occur even in the absence of a cutaneous burn. Zikria91 and Dowell25 and their coworkers as well as Traber and Herndon83 showed that the airway and lungs are initially damaged from incomplete products of combustion, most importantly aldehydes and oxides of sulfur and nitrogen. The injury is notable both for inhomog- eneous distribution within a patient and variable severity between patients. The physiochemical properties of the causative agent, amount of smoke inhaled, and preexisting diseases that might decrease the resistance of the recipient and determine the site and degree of injury. Because these patients often require intubation for airway protection, the synergy of inhalation injury with volutrauma can result in a severe ARDS-like state.
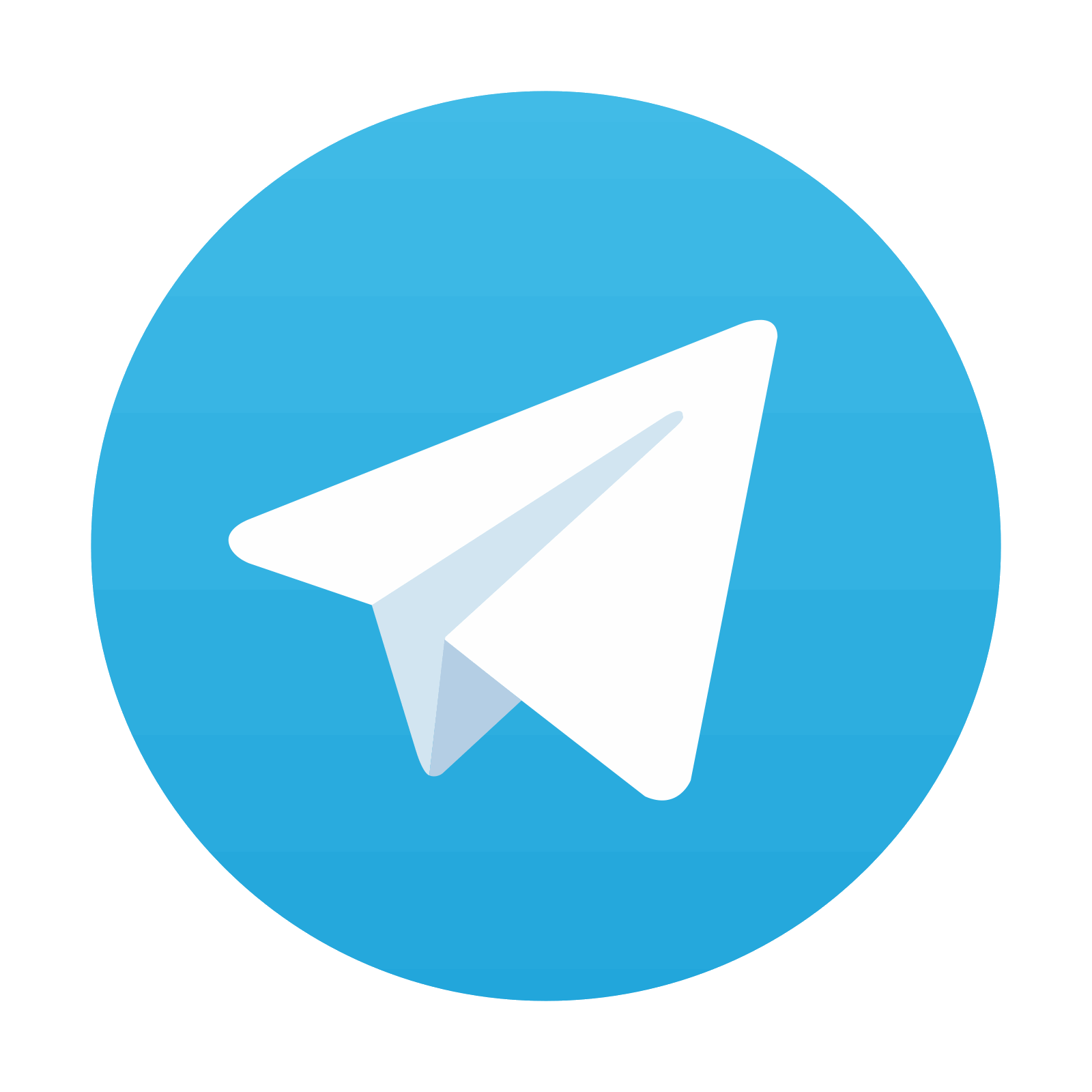
Stay updated, free articles. Join our Telegram channel
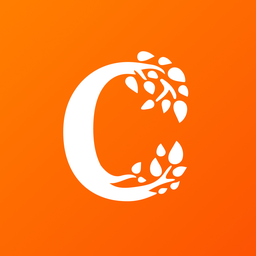
Full access? Get Clinical Tree
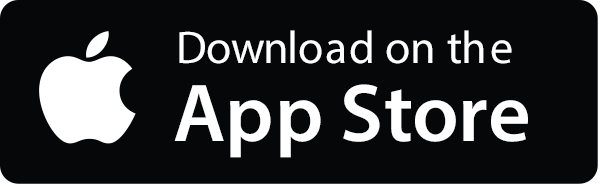
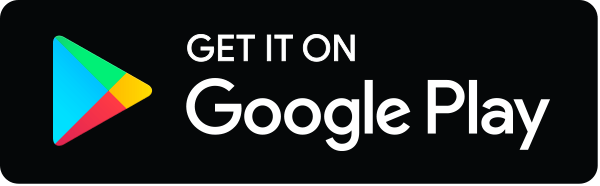