Fig. 27.1
ADInstruments Langendorff perfusion setup. Courtesy of ADInstruments
Several different methods for studying the isolated heart are possible, two of these methods are the Langendorff perfusion approach and the isolated working heart model [16, 17]. In the Langendorff system, constant pressure flow through an aortic cannula forces the aortic valve closed, and the perfusate passes through the coronary arteries without retrograde flow entering the left ventricle. This perfusion provides the myocardium with a physiologic solution, allowing the heart to beat without blood flowing through the four chambers. This method was named after Oscar Langendorff who, in 1895, was the first to describe an experimental model of an isolated mammalian heart as a technique to assess its required contractile activity. The advantage of the Langendorff perfusion method is that the measurement of EKG changes can be easily assessed, as well as measurement of metabolites that drain from the coronary sinus. Yet, the lack of flow in the left ventricle may limit its usefulness, i.e., minimal blood entering into the ventricle may promote clot formation, in turn, affecting the viability of the preparation. Additionally, the lack of flow in the ventricle may result in abnormal three-dimensional conformational changes in the heart that may cause coronary vascular compression. However, placement of a fluid balloon connected to a pressure transducer may allow for partial control of this problem, and may also be useful experimentally to assess changes in left ventricular function. Recent studies utilizing variations of the Langendorff working heart have adapted the setup for a range of different heart sizes. The classic hydrostatic afterload column is replaced with a centrifugal pump, allowing for easy adjustment and tight regulation of perfusion pressures, meaning the same setup can be used for various species or heart sizes [18].
The major disadvantage of the Langendorff preparation is that it does not eject the perfusate from the left ventricle and is therefore a non-work-producing model. This problem was initially overcome by Neely who used an isolated working heart which simulates physiological flow through the heart’s four chambers [19]. In this model, the perfusate is supplied by a cannula inserted into the left atrium; outflow through the left ventricle is monitored, while left atrial pressure or aortic pressure is controlled. This setup is considered ideal for the study of pressure and flow in the aorta as well as the left and right ventricles.
27.3.3 Additional Problems with Isolated Perfused Heart Models
Both types of isolated heart preparations have problems in common that should be considered when attempting to extrapolate results to the in vivo condition. First, the isolation process used for these models requires global myocardial ischemia (a period of no perfusion). Typically, once the organ is reperfused, baseline data (heart rate, left ventricular pressure, coronary blood flow) must be collected after a stabilization period to ensure relative viability of the preparation. Clearly, both the ischemic time and stabilization time may influence research outcomes. Therefore, any results obtained must be carefully analyzed with reference to the preparation’s baseline state as well as to the normal in vivo values, to avoid falsely attributing changes in cardiac function to the experimental protocol.
The composition of the perfusate can greatly impact the function and viability of the preparation in both of the aforementioned models. Early studies utilizing isolated heart models have employed whole blood as a perfusate [20]. However, significant problems with clotting and hemolysis may limit the time that the preparation remains viable. Saline compounds, which lack the potential for clotting and hemolysis, are considered useful alternatives to whole blood. However, such buffers have a lower colloid osmotic pressure and, coupled with the lower coronary vascular resistance, will typically result in progressive and severe edema; this results in interstitial edema formation and nonuniform perfusion. To extend the usefulness of the preparation, one can add osmotically active substances to the medium used for bathing and perfusing the preparation in an attempt to limit edema [21, 22]. Nevertheless, despite the technical difficulties associated with these models, isolated hearts have been used in research ranging from ischemia to transplant studies. For more details on isolated heart experimentation, the reader is also referred to Chap. 41.
27.4 Animal Models Used to Test Devices for Treatment of Valvular Disease
The significant morbidity and mortality associated with heart valve disease has produced a highly competitive market for manufactured prosthetic valves. Efforts to develop the ideal replacement heart valve have focused on producing a device that functions like the native valve (Table 27.1). To this end, certain basic principles of physics are fundamental in the design of mechanical valves, as evidenced by the evolution of various designs. The dynamics of blood flow through a tube with its specific viscosity is such that the flow is greatest in the center of the tube. Thus, any structure in the center of the valve (i.e., mechanical valve leaflets) will reduce the velocity through that valve (Fig. 27.2).
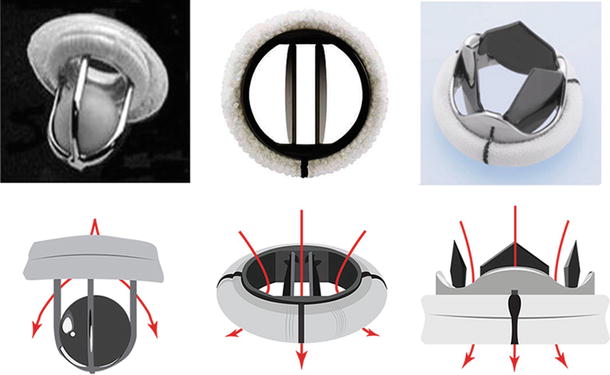
Table 27.1
Qualities of the ideal device for heart valve replacement
• Durable |
• Does not leak |
• Biologically inert |
• Nonthrombogenic |
• Facilitates laminar flow |
• Easily implanted by the surgeon |
• Quiet |
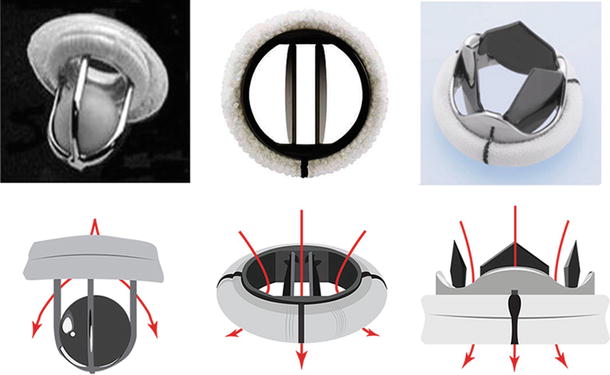
Fig. 27.2
Comparison of different mechanical valves with their flow characteristics. The evolution of the valve from the Starr-Edwards ball (left), the current standard bi-leaflet (center; St. Jude Medical, St. Paul, MN, USA), and a novel trileaflet design (right, Triflow Medical Inc.) currently in development. Below each valve is a stylized representation of the flow patterns reflecting the improvement in valve design
Guidelines for the design and testing of bio-artificial and/or mechanical heart valves have been established by the Center for Devices and Radiological Health of the Food and Drug Administration (FDA) and the International Organization for Standardization (ISO). The FDA has provided industry assistance in the form of guidance documents, advice, reporting, premarket approval, development of standards, and third-party reviews. Typically, prosthetic valve replacements are classified as either tissue (Fig. 27.3) or mechanical (Figs. 27.2 and 27.4), yet despite their common purpose, specific valve composition and function vary widely. Nevertheless, all valves must undergo performance-based testing to examine hydrodynamic performance (Table 27.2). For example, accelerated cyclic testing provides wear information, allowing for estimates of structural performance by providing data on fatigue, endurance limits, and damage tolerances of the valve.
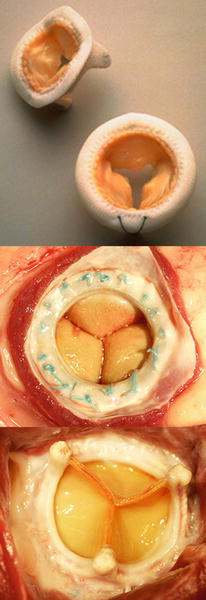
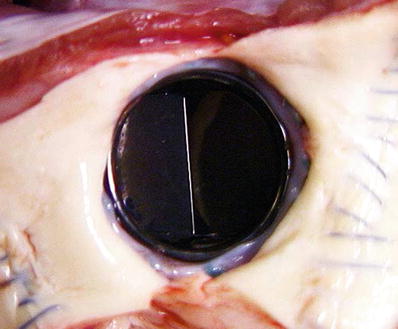
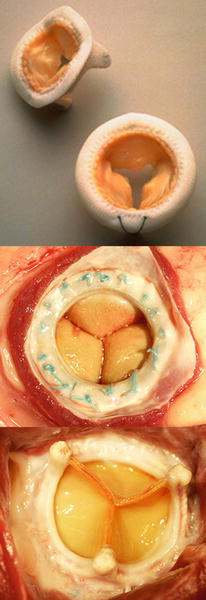
Fig. 27.3
(Top) The Medtronic Mosaic® stented tissue valve (Medtronic, Inc., Minneapolis, MN, USA). (Middle) Carpentier-Edwards Perimount Plus 6900P stented tissue valve, inflow aspect in the Mitral position in a sheep. (Bottom) Carpentier-Edwards Perimount Plus 6900P stented tissue valve, outflow aspect in the Mitral position in a sheep
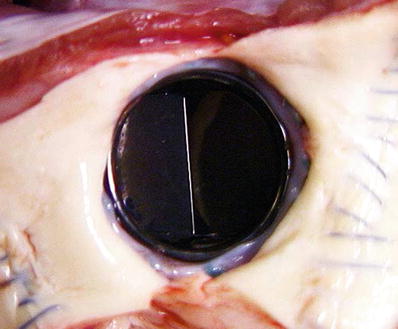
Fig. 27.4
Ovine model of a normal bileaflet mechanical valve implantation
Table 27.2
Mechanical valve fluid dynamic testing
• Forward flow testing |
• Backflow leakage testing |
• Pulsatile flow pressure drop |
• Pulsatile flow regurgitation |
• Flow visualization |
• Cavitation potential |
• Verification of the Bernoulli relationship |
Importantly, the FDA requires the demonstration of both efficacy and safety of prototype heart valve replacements prior to final approval for human implantation. This is based on the principle that additional technical and biological information can be gained by observing the valve in actual use. As a result, animal studies remain a crucial component in the overall evaluation of replacement heart valves [23]. To date, all investigational valves undergo a preclinical animal study with valve implantation in the orthotopic or anatomically normal position (with a required 20-week minimum period of evaluation). Specifically, the FDA looks for separate data from mechanical and biological valve studies. For example, mechanical valves generally place extreme shearing forces on the red blood cells and platelets, causing hemolysis and thrombosis that necessitate chronic anticoagulation after valve implantation. On the other hand, biologic valves place very low shear forces on the red blood cells and platelets, and thus there is no need for anticoagulation; however, they are sensitive to formation of calcium deposition, requiring the incorporation of some measures in their manufacturing that will attempt to prevent calcification after implantation. The lack of naturally occurring models of valve disease and the need for standardized models for FDA/ISO approval has led to the use of iatrogenic models of valve disease. To date, the ovine model has been used for producing a graded stenosis in the aortic and mitral valves by banding the aorta in young animals [24]. In contrast, aortic supravalvular stenosis, as well as aortic valvular stenosis, has been commonly induced in the canine model [25, 26]. Additionally, induction of mitral valve regurgitation in the canine is possible by placement of a shunt [27] or by transection of the chordae tendineae. Interestingly, experiments have also been performed to induce stenosis or regurgitation in the tricuspid and pulmonic valves [28]. However, most valve implantation studies approved for human use are completed in normal animals and their primary goals are to strictly examine valve performance (Fig. 27.4). A thorough understanding of the background and natural history of heart valve disease in standard laboratory animal species is needed to ensure that spontaneous valve lesions are not misinterpreted as treatment related [29].
A principal advantage of employing the canine model is the large amount of background information available in the cardiovascular surgical literature. Historically, the dog was considered to be the gold standard for both acute and chronic models of valve replacement that was accepted by the FDA. Early success with the canine model in valve replacement identified the need for minimizing the risk of surgical infection at the time of prosthesis implantation. Specifically, the use of preoperative parenteral and postoperative topical antibiotics, strict sterile techniques, minimum numbers of operative arterial and venous lines, and short cardiopulmonary bypass times were all noted parameters to minimize the risk of bacterial valve implant seeding [30].
As described in Chap. 6, the anatomy of the porcine heart is most similar to that of the human heart in regards to the conduction system, coronary arteries, blood supply to the conduction system, and great vessels. In addition, the coagulation cascade of the swine is quite similar to that of humans. Despite these advantages, several problems have been identified in using this model for valvular research. First, the porcine heart is extremely sensitive to anesthesia, and surgical manipulation often results in postsurgical complications, arrhythmias, and/or death. Second, the growth of young swine is rapid, resulting in heart size and physiological flow that is not constant over required follow-up periods of several months; yet, if you want to investigate the effects of heart growth on a device, using the swine may be beneficial. Specifically, these alterations often result in fibrous sheathing and obstruction of the valve orifice, thrombus formation, or dehiscence (separation) of the sewing cuff from the native annulus. Finally, significant bleeding complications due to application of anticoagulation therapy and poor survival have limited the use of the pig in studying valve-related thrombosis [31]. A recent porcine model for aortic valve sclerosis was able to mimic early human aortic valve disease by feeding swine a high-fat/high-cholesterol diet. This study showed the efficacy of modifying certain factors in a study, allowing for animal models to mimic changes seen in humans [32].
The ovine model is currently accepted as the gold standard for valve replacement using defined survival surgeries that meet FDA requirements. Normal cardiovascular physiological parameters of sheep approximate those of humans in blood pressure, heart rate, cardiac output, and intracardiac pressure [33]. In addition, the anatomy of the adult heart provides valve orifice diameters that are similar to humans [34]. The use of animals of similar age and weight (8–12 months, 30–40 kg) allows for the testing of replacement valves using a single orifice size for comparison of valve performance to an appropriate standard. Although the heart and vessels are small in animals within this weight range, the sheep’s relatively large left and right atria allow for straightforward surgical approaches to either the mitral or tricuspid valves.
In general, sheep as experimental animals allow for easy handing and long-term husbandry. Furthermore, juvenile sheep grow at a rate that does not cause excessive mitral or aortic stenosis during the postimplantation test periods, as compared to the porcine model [31]. However, specific attention to gastric decompression, perioperative antibiotics, sterile techniques, and minimally invasive interventions in the postoperative period will all increase the success of valve implantation studies in the ovine model [35].
27.4.1 Animal Models of Atrial Fibrillation for Preclinical Valve Testing
Given the increasing number of patients afflicted with atrial fibrillation worldwide, an animal model of the disorder is needed to predict valvular function and its effects on the natural course of the disease. For example, in one study, atrial fibrillation was associated with morbidity secondary to stroke (13 %) and congestive heart failure (24 %) despite anticoagulant treatment and independent of New York Heart Association (NYHA) functional classification, type of surgery, coronary artery disease, history of coronary artery bypass graft surgery, or other cardiac risk factors [10]. Previous research has uncovered a number of cardiovascular structural and electrophysiological alterations associated with atrial fibrillation [23–26]. More specifically, the fibrillating heart will have a shorter refractory period at the right atrial appendage, shorter action potential duration, electrophysiological remodeling, and changes in gene expression [24, 26]. Myocardial remodeling leading to atrial enlargement appears to be a direct result of atrial fibrillation. From a structural standpoint, the fibrillating left atrium is larger, has relative stasis of blood particularly in the atrial appendage, and fails to give the “atrial kick” which comprises approximately 20 % of ventricular filling. These characteristics also explain the increased thromboembolic risk and decreased cardiac output associated with atrial fibrillation.
27.4.2 Pacing-Induced Atrial Fibrillation
Control of the heart beat using electrical stimulation is usually achieved using an intracardiac or transesophageal approach. Intracardiac pacing, subdivided into burst pacing and continuous pacing, is the most commonly used procedure for the induction of atrial fibrillation in the sheep model and in animal models overall. Rapid atrial pacing is the most common method of inducing atrial fibrillation for in vivo investigation. The transesophageal approach to pacing represents another possibility; however, it is used in humans and animal models primarily in the detection and assessment of irregular cardiac rhythms and coronary artery disease [36]. It should also be noted that implantable systems offer another alternative for the delivery of right atrial rapid pacing in order to induce atrial fibrillation in conscious animals [37].
27.4.3 Pharmacologic-Induced Atrial Fibrillation
Administration of catecholamines and acetylcholine perfused through the sinoatrial nodal artery can induce atrial fibrillation. Isoproterenol (nonselective beta-adrenergic agonist) and adrenaline (alpha-and beta-adrenergic agonist) induce atrial fibrillation in dogs [38]. Atropine treatment prevented catecholamine-mediated atrial fibrillation, indicating a critical role of cholinergic tone in these atrial fibrillation episodes. Acetylcholine-mediated atrial fibrillation is facilitated by isoproterenol, which decreases the threshold of acetylcholine concentration required for atrial fibrillation induction and increases the atrial fibrillation duration. The focal delivery of these agents into atrial tissues can also cause episodic fibrillation.
27.4.4 Other Potential Atrial Fibrillation Models
Given that many genes are associated with cardiac contractility, it is reasonable to postulate that genetic engineering may have a potential role in the development of an atrial fibrillation model. The first important advance in this direction has been the identification of a genetic locus for familial atrial fibrillation on chromosome 10q22-q24 [39]. The discovery that stem cell-derived cardiomyocytes have an intrinsic arrhythmic potential further leads to the question whether stem cell therapy could be the basis for a model of atrial fibrillation [40].
27.5 Animal Models in Myocardial Ischemia
Despite great advances in treatment options, atherosclerotic coronary vascular disease remains one of the leading causes of death worldwide. As a result, this disease continues to be an active area of cardiovascular research. Originally defined by the Greeks as a lack of blood flow, the modern definition of ischemia emphasizes both the imbalance between oxygen supply and demand as well as the inadequate removal of waste products. Impaired oxygen delivery causes a reduction in oxidative phosphorylation, resulting in myocardial dependence on anaerobic glycolysis for the production of high-energy phosphates. This shift in metabolism produces excess lactate which then accumulates in the myocardium. As impaired ATP production and local tissue acidosis prevails, there is a resultant decline in cardiac contractility. Ultimately, if ischemia is not reversed, myocardial infarction occurs with permanent cellular loss and impaired cardiac function. Multiple experimental techniques have been developed for the study of cardiac ischemia. Currently, scientists consistently use isolated myocytes to examine single cell responses, while isolated perfused hearts and whole animal models allow for a better understanding of the whole organ responses. Regardless of the model type, experimental animals remain a crucial tool in the area of research.
27.5.1 Experimental Methods for Creating Ischemia
The ideal model for investigations of ischemic myocardium would theoretically be the intact chronically instrumented awake animal, as acute surgical trauma and anesthetic agents both depress cardiac function [2]. The conscious animal model also has the advantage that it can be used in studies requiring physiological stress, e.g., stress produced by exercise. However, the high cost of the implanted transducers and probes as well as difficulties with measurement techniques often preclude the use of such an approach. To date, the majority of studies use anesthetized animal models for the study of ischemia in either closed or open chest models. Closed chest models have the advantage that tissue trauma is minimized, but in such models, direct access to the heart for metabolite measurement is a major limitation. In contrast, the open chest preparation has the advantage that regional function and metabolism can be studied in detail. The open chest models suffer from drawbacks that include a greater susceptibility to temperature variations and a potential for surgical trauma that may considerably alter cardiac function (Fig. 27.5).
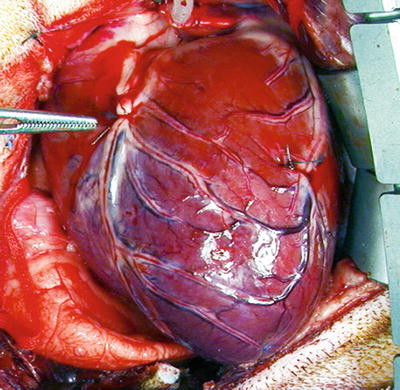
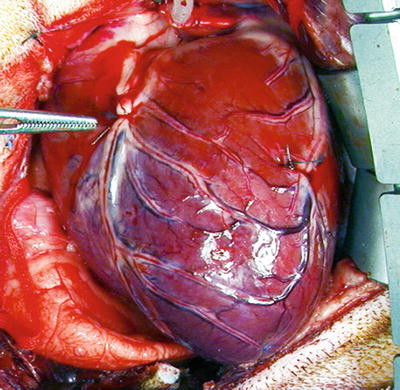
Fig. 27.5
Ligated left anterior descending coronary artery (adjacent to forceps) in an open chest canine model
Multiple techniques have been used to create models of myocardial ischemia for research purposes, depending on whether the desired occlusion is to be permanent, temporary, or progressive. Methods to produce complete permanent occlusions include surgical coronary artery ligation or radiological embolization with microparticles. Furthermore, permanent or temporary partial coronary occlusions are commonly induced by ligation, balloon occlusion, or clamping. Typically, models of progressive coronary artery occlusions use either balloon/catheter occlusion or ameroid constrictors (Fig. 27.6). Regardless of the method chosen, the researcher must be aware that the concentric experimental lesions that are created will differ from those of naturally occurring atherosclerotic coronary vascular disease which are typically eccentric. Normally, such eccentric stenoses remain vasoactive and are capable of altering coronary blood flow by changing their lumen diameter. It should be noted that no such vasoactivity remains in experimentally created concentric lesions which will prohibit humoral agents from altering regional coronary flow (Fig. 27.7).
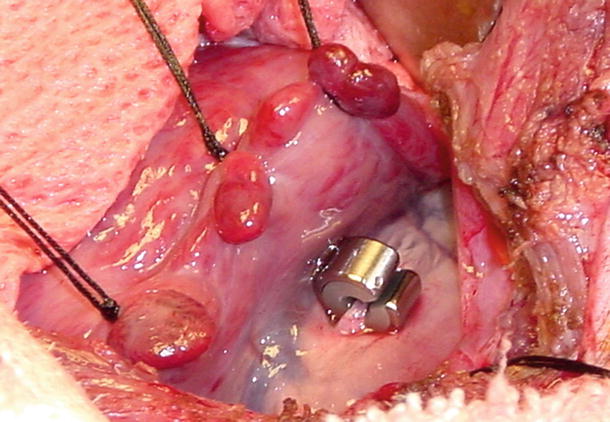
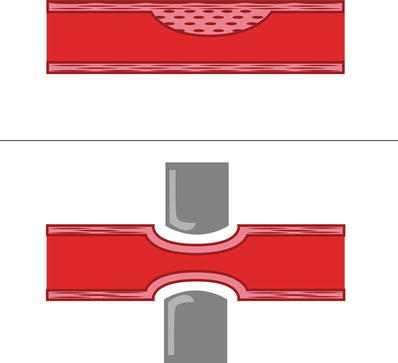
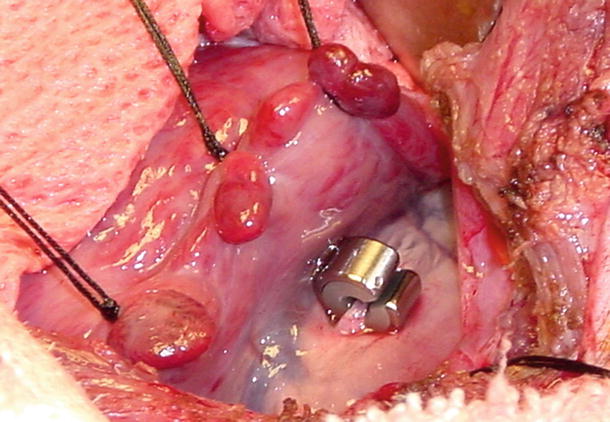
Fig. 27.6
Ameroid occluder in the canine model . Photo courtesy of Michael Jerosch-Herold and Cory Swingen
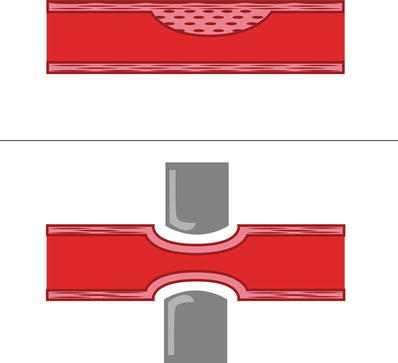
Fig. 27.7
(Top) Example of an eccentric vascular constriction as with coronary artery disease. (Bottom) Concentric lesion as created by experimental ligation or ameroid occlusion
Experience has shown that an induced occlusion of the left anterior descending coronary artery is favored over that in the left circumflex coronary artery for the production of regional myocardial ischemia. It is generally accepted that the occlusion of the left anterior descending coronary artery results in a larger area of myocardial ischemia, and therefore greater impairment of global left ventricular function. However, estimates of infarction size alone have not correlated well with ventricular function [41]. Thus, it has been demonstrated that for the same amount of ischemic myocardium, the compensatory increase by the nonischemic myocardium is different for the left anterior descending coronary artery and the left circumflex coronary arteries [42]. Therefore, in an ideal model, both infarct size and its location must be similar in order to achieve the same degree of impairment in left ventricular global function. If possible, one should also estimate the ischemic area at risk due to an imposed occlusion, e.g., with imaging or the use of dyes.
27.5.2 Localizing and Quantifying Myocardial Ischemia
Blood samples collected from the coronary sinus or from a regional coronary vein are commonly obtained and used for metabolic studies. Yet, such results must be interpreted with the knowledge that these samples include blood from adjacent noninjured myocardium. The use of coronary venous samples for studying metabolism is decreasing because of new approaches using microdialysis, MRI, nuclear magnetic resonance spectroscopy, and positron emission tomography [43–45].
The size and location of myocardial infarction can be determined by Triphenyltetrazolium chloride (TTC) staining , which has been the gold standard for quantifying the extent of myocardial infarction in pathological specimens [46] (Fig. 27.8). In addition, the assessment of localized tissue blood flow using microspheres (radioactive or colored) remains another important standard. However, newer noninvasive methods of determining blood flow in the live animal that allow for repeated follow-up determinations are being developed and improved upon, including spectroscopy and MRI.
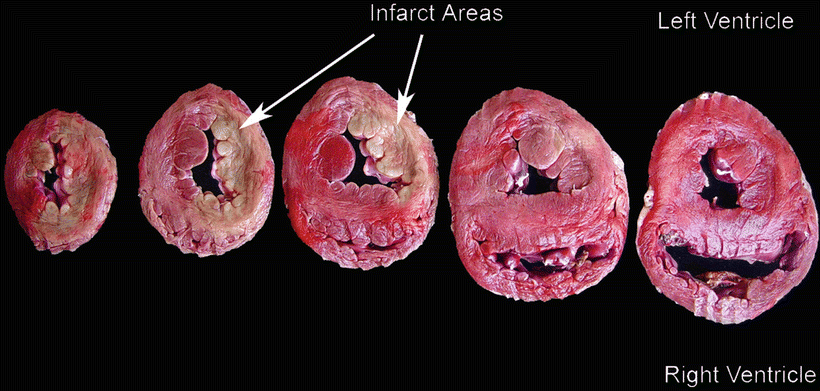
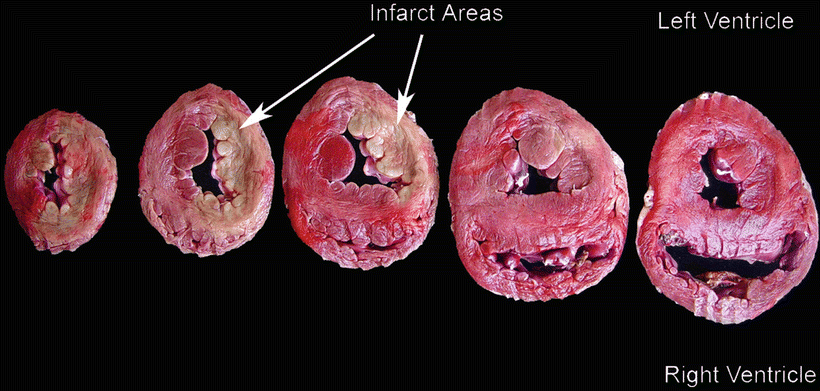
Fig. 27.8
Triphenyltetrazolium chloride (TTC) staining in canine infarct model showing pallor of myocardium (infarcts, arrows) in left anterior coronary artery distribution
27.5.3 Specific Animal Models for Ischemia Investigations
Both large and small animal models have been developed for the study of myocardial ischemia. Advantages of large animal models are their similarity in physiology to humans and ease of instrumentation, and disadvantages include significantly greater care and cost issues that may make small animal models more attractive, particularly when large numbers of animals are required to achieve significant statistical power [47].
The dog has been the most frequently utilized species for in vivo studies of chronic ischemia because dogs have a well-developed coronary collateral circulation, similar to humans with chronic ischemia (progressive heart failure). Furthermore, dogs are easy to handle and lack significant growth as adults, which allows long-term follow-up. However, the significant variability in coronary collateral circulation may hamper efforts to create consistent sizes of ischemic regions between animals, or may result in a minimized ischemic zone.
The pig heart is closer to the relatively healthy human heart with limited collateral blood flow; this makes the swine heart ideal for acute ischemia studies. However, long-term follow-up using the swine model, in general, is considered problematic; if juvenile animals are utilized, significant changes in animal weight will result in both increased difficulties with handling as well as alterations in basic cardiac physiology. More specifically, in consideration of heart to body weight ratios, in a healthy person the ratio is about 5 g/kg, for pigs weighing between 25 and 30 kg, the ratio is similar to that in humans, but for animals exceeding 100 kg, it is only half that value [42]. Importantly, such ratio changes must be considered when interpreting experimental results.
Small animals have also been used as models for investigations of regional myocardial ischemia. However, it has been established that the collateral circulation of the rat is sparse and that of the rabbit may show intraspecies differences [48]. In turn, the guinea pig has such an extensive collateral network that normal perfusion is maintained after a coronary artery occlusion and often infarction does not develop. Another problem with using these animals is that the small vessel diameters may delay or prevent instantaneous reperfusion following transient vessel occlusion, which is further complicated by the inability to make quantitative assessments of coronary blood flow in these small vessels to verify reperfusion. Nevertheless, the use of small animal models for studying myocardial ischemia remains important, including recent studies using stem cells for treatment. See also Chap. 6 for additional details on the comparative coronary circulations .
27.6 Animal Models in Heart Failure and Transplantation
Alexis Carrel reported the first heterotopic transplantation (Table 27.3) of a canine heart connected to the neck vessels of another dog in 1905, but the transplant succumbed to massive clotting and the animal survived for only 2 h. Many years later, Richard Lower and Norman Shumway perfected an orthotopic transplantation technique in the canine and achieved heart graft survivals of up to 21 days. Translation of this research to clinical practice was first performed by Christiaan Barnard in 1967, but acceptable graft survival required further studies in animal models to overcome rejection by the host immune system.
< div class='tao-gold-member'>
Table 27.3
Definition of graft types
Graft Type | Definition |
---|---|
Autograft | Transplant from one site to another in the same individual |
Isograft | Transplant from a donor to a genetically identical individual (monozygotic twin) |
Syngraft | Transplant from a donor to a recipient with no detectable genetic difference (inbred strain) |
Allograft (homograft) | Transplant from a donor to a genetically different individual of the same species |
Xenograft (heterograft) | Transplant from a donor to a recipient of another species |
Only gold members can continue reading. Log In or Register a > to continue
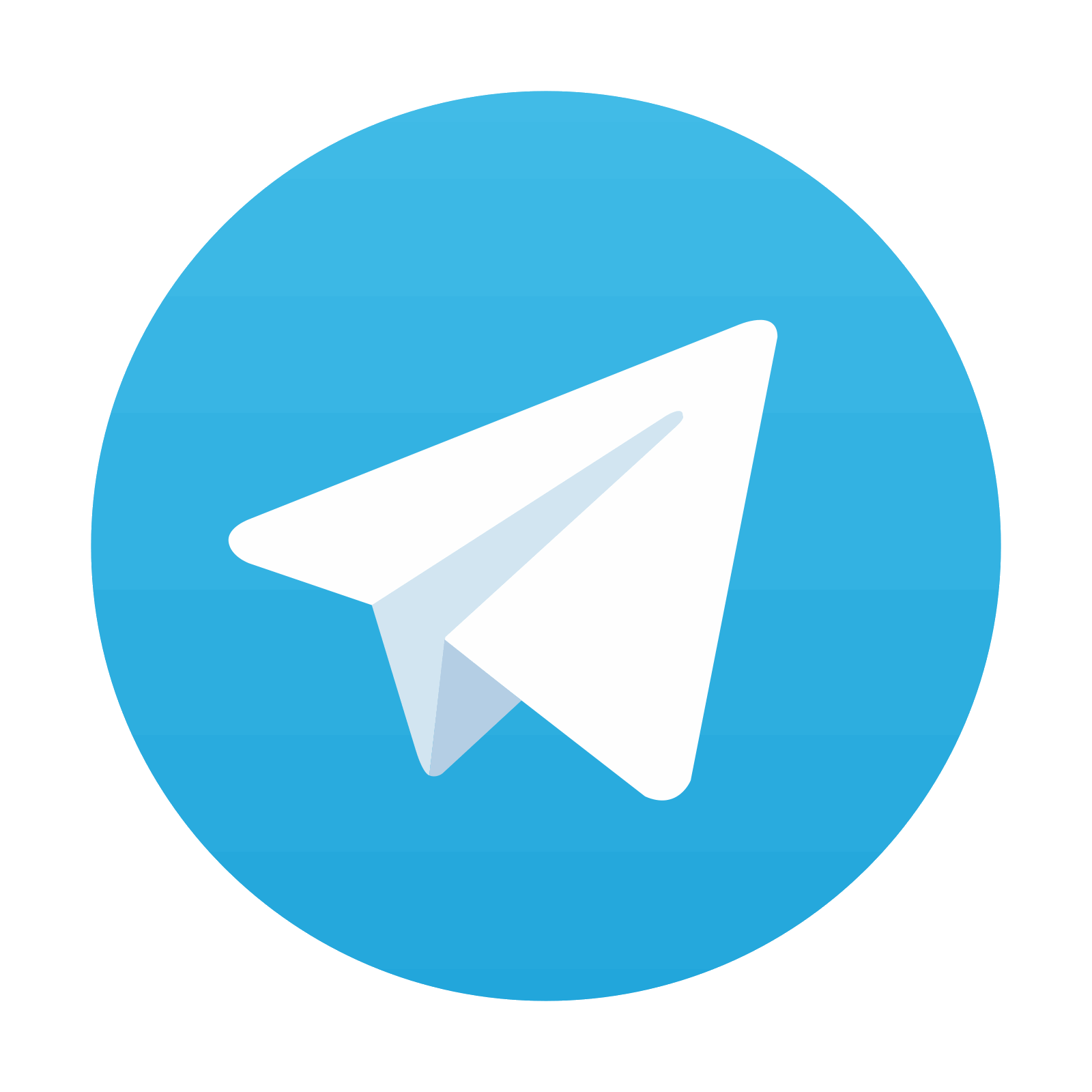
Stay updated, free articles. Join our Telegram channel
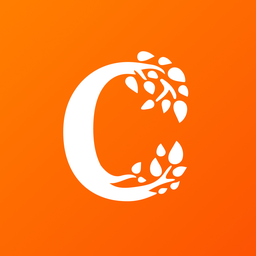
Full access? Get Clinical Tree
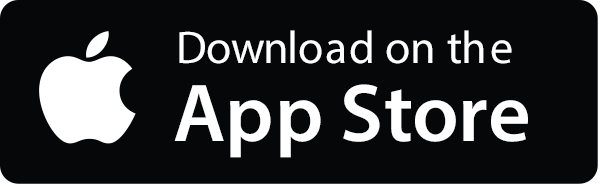
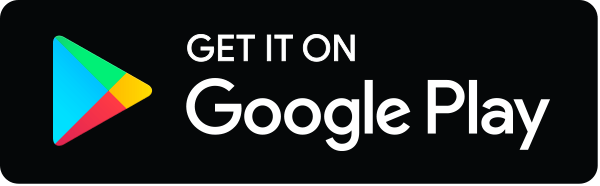