Introduction
The lung has two essential, interdependent functions. One function is ventilation-perfusion matching to deliver oxygen to the body and to remove carbon dioxide that is produced by the body ( Fig. 1-1 ). The second function is host defense against the onslaught of airborne pathogens, chemicals, and particulates. These essential functions are emphasized through the gross, subgross, histologic, and ultrastructural determinants of respiratory gas exchange in the normal human lung. Secondary functions of the lung also are important, such as surfactant synthesis, secretion, and recycling; mucociliary clearance; neuroendocrine signaling; and synthesis and secretion of a myriad of molecules by its epithelial and endothelial cells. The diversity of secondary functions emphasizes the importance of the lung in homeostasis. The chapter finishes with comparison of the lung of mice and humans, an important subject given the widespread use of murine models in lung research. to provide views of lung movements related to changes in tidal volume, airway pressures, and respiratory rate.

Gross and Subgross Organization
The position of the lungs in the chest and in relationship to the heart is shown in Fig. 1-2 . Figure 1-2A shows a midfrontal section through the thorax of a frozen human cadaver. Figure 1-2B shows a posterior-anterior chest radiograph of a normal human at functional residual capacity (FRC). The two illustrations represent the extremes of the approaches to lung anatomy. The cadaver lung (see Fig. 1-2A ) shows the gross anatomic arrangements and relationships. The main distortion is that the lungs are at low volume. The vertical height of the lungs is only approximately 18 cm, which is well below that at FRC (see Fig. 1-2B ). The diaphragm is quite elevated in Figure 1-2A , and is approximately 5 cm higher than its end-expiratory position in life. Another distortion is the abnormally wide pleural space; however, this fixation shrinkage artifact serves as a useful reminder that the lung is not normally attached to the chest wall. In life the separation between the parietal and visceral pleuras is only several micrometers. The chest radiograph (see Fig. 1-2B ) shows that the vertical height of the lung at FRC is approximately 24 cm, with the level of the bifurcation of the pulmonary artery approximately halfway up the lungs. The diaphragm is lower and flatter than in the cadaver.

In life the human lungs weigh 900 to 1000 g, of which nearly 40% to 50% is blood. At end-expiration, the gas volume is approximately 2.5 L whereas, at maximal inspiration, it may be 6 L. Thus overall lung density varies from 0.30 g/mL at FRC to 0.14 g/mL at total lung capacity. But the density of the lung is not distributed uniformly, being approximately 1 g/mL near the hilum and 0.1 g/mL peripherally. If one likens each lung to a half cylinder, more than 50% of all the lung’s alveoli are located in the outer 30% of the lung radius (hilum to chest wall). This is why the peripheral portion of the lung appears relatively empty in the chest radiograph (see Fig. 1-2 ). Variability in density also exists from top to bottom. In Figure 1-2 the blood vessels are more distended in the lower lung fields. The increasing distention of vessels from apex to base also illustrates the increase in vascular distending pressures at the rate of 1 cm H 2 O/cm height down the lung.
The disposition of the various tissues that constitute the lung is summarized in Table 1-1 . An amazing point is how little tissue is involved in the architecture of the alveolar walls. But this is as it should be because the major physical problem of gas exchange is the slowness of oxygen diffusion through water. Thus the alveolar walls must be extremely thin. In fact, the thickness of the red blood cell forms a substantial portion of the air-blood diffusion pathway. Advantage was taken of this fact to separate the carbon monoxide diffusing capacity measurement into two components: the capillary blood volume and the membrane diffusing capacity. (For a discussion of diffusing capacity, see Chapters 4 and 25 .)
Component | Volume or Mass (mL) | Thickness (µm) | Reference No. |
---|---|---|---|
Gas | 2400 | ||
Tissue | 900 | ||
Blood | 400 | ||
Lung | 500 | ||
Support structures | 225 | ||
Alveolar walls | 275 | ||
Epithelium | 60 | 0.18 | |
Endothelium | 50 | 0.10 | |
Interstitium | 110 | 0.22 | |
Alveolar macrophages | 55 |
The lung has two well-defined interstitial connective tissue compartments arranged in series, as described by Hayek ( Fig. 1-3 ). These are the parenchymal (alveolar wall) interstitium and the loose-binding (extra-alveolar) connective tissue (peribronchovascular sheaths, interlobular septa, and visceral pleura). The connective tissue fibrils (collagen, elastin, and reticulin) form a three-dimensional basket-like structure around the alveoli and airways ( Fig. 1-4 ). This basket-like arrangement allows the lung to expand in all directions without developing excessive tissue recoil. Because the connective tissue fibrils in the parenchymal interstitium are extensions of the coarser fibers in the loose-binding connective tissue, stresses imposed at the alveolar wall level during lung inflation are transmitted not only to adjacent alveoli, which abut each other, but also to surrounding alveolar ducts and bronchioles, and then to the loose-binding connective tissue supporting the whole lobule, and ultimately to the visceral pleural surface (see Fig. 1-3 ). These relations become more apparent in certain pathologic conditions. For example, in interstitial emphysema, air enters the loose-binding connective tissue and dissects along the peribronchovascular sheaths to the hilum and along the lobular septa to the visceral pleura. Interstitial pulmonary edema liquid enters and moves along the same interstitial pathways ( Fig. 1-5 ).



The bulk of the interstitium is occupied by a matrix of proteoglycans ( Fig. 1-6 ). Proteoglycans constitute a complex group of gigantic polysaccharide molecules (≈30 different core proteins, with great diversity of glycosaminoglycan side chains) whose entanglements impart a gel-like structure to the interstitium. That structural role, although essential, is not the sole role of these important molecules. A growing view is emerging of the lung’s extracellular matrix components as regulators of lung physiology, helping in determining epithelial cell phenotype; binding of and subsequent signaling by cytokines, chemokines, and growth factors; and mediating cell proliferation, migration, differentiation, and apoptosis. In disease states, degradation products of extracellular matrix components may activate the Toll-like receptor pathways (see later discussion); thus the degradation products may serve as endogenous sentinels of tissue damage and initiators of innate immune responses. Within this gel-like interstitium reside several varieties of interstitial cells (contractile and noncontractile interstitial cells, mast cells, plasma cells, and occasional leukocytes). The remainder of the interstitium is composed of laminin, collagens, elastin and reticulin fibrils, fibronectin, and tenascin (see Fig. 1-6 ).

Airways
The airways, forming the connection between the outside world and the terminal respiratory units, are of central importance to our understanding of lung function in health and disease. Intrapulmonary airways are divided into three major groups: bronchi ( Fig. 1-7 ), bronchioles (including the terminal bronchioles) ( Fig. 1-8 ), and respiratory bronchioles ( Fig. 1-9 ; see Fig. 1-8 ). By definition, bronchi have cartilage in their wall, whereas bronchioles do not. Respiratory bronchioles serve a dual function as airways and as part of the alveolar volume (gas exchange).



The anatomic dead space, as measured by the single-breath nitrogen dilution technique, is approximately 30% of each tidal volume. Anatomically this dead space is accounted for principally by the volume of the extrapulmonary (upper) airway, including the nasopharynx and trachea, and the intrapulmonary bronchi. The trachea and bronchi are cartilaginous, do not change shape significantly with ventilation, and do not participate in gas exchange. Bronchioles, approximately 1 mm in diameter or less, have no cartilage and are exceedingly numerous and short. They consist of approximately five branching generations and end at the terminal bronchioles. In contrast to the bronchi, the bronchioles are tightly embedded in the connective tissue framework of the lung and therefore enlarge passively as lung volume increases. Histologically the bronchioles down to and including the terminal bronchioles ought to contribute approximately 25% to the anatomic dead space. In life, however, they contribute little because of gas-phase diffusion and mechanical mixing in the distal airways resulting from the cardiac impulse. By definition, the respiratory bronchioles and alveolar ducts participate in gas exchange and thus do not contribute to the anatomic dead space. The volume of the respiratory bronchiole-alveolar duct system is approximately one third of the total alveolar volume, and it is into this space that the fresh-air ventilation enters during inspiration.
Most airway resistance resides in the upper airway and bronchi. Normally the large airways maintain partial constriction. The minimal airway diameter in the human lung, approximately 0.5 mm, is reached at the level of the terminal bronchioles; succeeding generations of exchange ducts (respiratory bronchioles and alveolar ducts) are of constant diameter (see Fig. 1-9 ). The functional significance of centralized resistance is that the terminal respiratory units (the physiologic alveoli) are regionally ventilated chiefly in proportion to their individual distensibilities (compliances) because most of their airway resistance is common. This is demonstrated normally by the finding that regional lung ventilation is dependent upon the initial volumes of the alveoli. Terminal respiratory units toward the top of the lung, which are more expanded at FRC, do not receive as great a share of the inspiratory volume as do the terminal respiratory units near the bottom of the lung.
The balance between anatomic dead space volume, for which the airway diameter ought to be as small as possible to maximize efficient alveolar ventilation (dead space–to–tidal volume ratio), and airflow resistance, for which the airway diameter ought to be as large as possible to minimize the work of breathing, requires a compromise. Normally, anatomic dead space is not maximal, nor is resistance minimal. In disease, by contrast, airways may narrow ( Fig. 1-10 ), which increases resistance.

The cellular complexity of the airways is indicated by the nearly 50 distinct cell types found there, at least 12 of which are epithelial cells on the airway surface. Nearly half of the epithelial cells in the normal human airway are ciliated at all airway generations ( Fig. 1-11 ) down to bronchioles ( Fig. 1-12 ). Cilia move the superficial liquid lining layer ( Fig. 1-13 ; see Fig. 1-11 ) continually toward the pharynx from deep within the lung. As the superficial lining liquid moves centripetally, the total perimeter of the airways decreases markedly. If the lining liquid volume remained constant, the liquid layer ought to thicken but this does not happen, suggesting that much of the liquid is reabsorbed during its ascent along the airways.



The presence of apical junctional complexes between airway epithelial cells (see Fig. 1-13 ) has important functional implications for metabolically-regulated secretion into and absorption of electrolytes and water from the lining liquid. Apical junctional complexes consist of three elements: zonula occludens (tight junction), zonula adherens , and macula adherens (desmosome). Tight junctions subserve two important functions: (1) restriction of passive diffusion by blocking the lateral intercellular space and (2) polarization of cellular functions (ion and water transport) between the apical and basolateral membranes. Polarization of chloride and sodium transport allows the airway epithelium either to secrete or to absorb ions, with associated water movement.
Trapping of foreign material, such as particulates or bacteria, is accomplished by mucins. Mucins are complex glycoproteins that form gels, exemplified by MUC5A. MUC5A is present in the lung of humans. Other mucins (e.g., MUC5B, MUC7) become expressed by airway epithelial cells in diseases, such as cystic fibrosis. In that disease, MUC5B is produced by airway epithelial cells. Normally MUC5B is produced by airway glandular cells, but, in a variety of pulmonary diseases, its cell source is expanded.
Glands are limited to the submucosa of the bronchi. Airway glands secrete water, electrolytes, and mucins into the lumen ( Fig. 1-14 ; see Fig. 1-7 ). Studies of the regulation of secretion in vivo and in vitro have shown that release can be modulated by neurotransmitters, including cholinergic, adrenergic, and peptidergic transmitters, and by inflammatory mediators such as histamine, platelet-activating factor, and eicosanoids. Goblet cells, which are mucin-secreting epithelial cells, also are present at most airway levels (see Fig. 1-13 ). Goblet cells decrease in number peripherally, normally disappearing at terminal bronchioles. The absence of airway glands and goblet cells distal to ciliated epithelial cells makes sense because that arrangement should minimize the flow of mucus backward into alveolar ducts and alveoli.

Lymphocytes are frequently seen intercalated between airway epithelial cells ( Fig. 1-15 ; see Fig. 1-11 ). These cytotoxic T lymphocytes undergo IgA class antibody responses. T and B lymphocytes also accumulate in the lamina propria beneath the airway epithelium.

Although most foreign material and immunologic stimuli are carried up the airways by mucociliary action, some are cleared by the lymphatics (discussed at the end of this chapter). In addition, lymphoid tissue is located in the lungs. Patches are distributed along the tracheobronchial tree (see Fig. 1-7 ) and, to a lesser extent, along the blood vessels. These patches apparently develop in response to antigenic stimulation because they are not present at birth in humans or in germ-free animals. Lymphocytes in these aggregates are principally B cells that express mainly IgA immunoglobulins. The presence of lymphocytes along the airways provides a reminder that the respiratory system is constantly challenged by airborne immunologic stimuli. The tracheobronchial lymphoid tissue, including bronchus-associated lymphoid tissue, appears to provide an important locus for both antibody-mediated and cell-mediated immune responses. Another important locus of immune response is provided by the epithelial cells that line the airways and constitute the airway glands. Their importance stems from production of Toll-like receptors, whose role is identification of pathogen-associated molecular patterns. Activation of Toll-like receptors leads to downstream signaling cascades that are involved in mucin production, leukocyte recruitment, antimicrobial peptide production, wound repair, and vascular formation.
Some of the other cells associated with the airways are smooth muscle cells, mast cells, basal cells, and club cells (Clara). Smooth muscle cells form circular bands around the airway epithelium as far peripherally as the respiratory bronchioles (see Figs. 1-7 and 1-8 ). Smooth muscle tone is altered by the autonomic nervous system and by mediators released from mast cells, inflammatory cells, and neuroendocrine cells. During normal breathing, slight tonic contraction of small airway smooth muscle cells and reflex contraction of the larger airways stiffens them against external compression, as may result from forced expiration or coughing. The effector of these responses is the parasympathetic limb of the autonomic nervous system (vagus nerves). Therefore excessive vagal input causes severe contraction of airway smooth muscle and increases mucus secretion by submucosal glands, both of which limit airflow through conducting airways by decreasing airway lumen diameter and increasing airway resistance.
Mast cells in the human lung contain membrane-bound secretory granules that are characteristically filled by scrolled, crystalline, or particulate inclusions ( Fig. 1-16 ). These granules contain a host of inflammatory mediators, including histamine, proteoglycans, lysosomal enzymes, and metabolites of arachidonic acid. Not only can these mediators induce bronchoconstriction, they can also stimulate mucus production and induce mucosal edema by increasing permeability of bronchial vessels.

Basal cells are located along the basal lamina of airways (see Fig. 1-13 ). These small epithelial cells have been classically thought to be precursor cells for other airway epithelial cells, including ciliated cells. However, more recent experiments suggest that columnar secretory cells or club cells may also differentiate into ciliated epithelial cells following tissue injury.
Club cells (Clara), prominent in the terminal airways, are interspersed among the ciliated epithelial cells, are nonciliated, and have large apical granules (see Fig. 1-15 ). Club cells have at least four functions in the lung. One function is serving as progenitor cells for themselves and for ciliated epithelial cells. A second function is xenobiotic metabolism via the cytochrome P-450 monooxygenase system. A third function is secretion: club cells are a source of surfactant proteins (SPs; SP-A, -B, and -D) and also of lipids, proteins (club cell 10-kDa protein), glycoproteins, and modulators of inflammation (leukocyte protease inhibitor and trypsin-like protease). A fourth function is liquid balance by influencing ion channels.
Bronchial Circulation
The trachea (and esophagus), main-stem bronchi, and pulmonary vessels into the lung (see Fig. 1-1 ), as well as the visceral pleura in humans (see “ The Pleural Space and Pleuras ” toward the end of this chapter), are supplied by the bronchial (systemic) circulation. Measurements of bronchial circulation, by microsphere studies in animals, indicate that flow is 0.5% to 1.5% of cardiac output and is predominantly to the large airways. The bronchial arteries arborize into bronchial capillaries that form a network in the lamina propria, in the submucosa, and in the region external to the cartilage of bronchi, as well as in the lamina propria of neighboring pulmonary arteries. Venous blood from the trachea and large airways enters bronchial venules, which converge to form bronchial veins that drain into the azygos or hemiazygos veins. Thus a substantial part of bronchial blood flow returns to the right side of the heart. Deeper in the lung, however, bronchial blood passes via short anastomotic vessels into the pulmonary venules, thus reaching the left side of the heart to contribute to the venous admixture.
The bronchial circulation has enormous growth potential, which is in contrast to the pulmonary circulation, which after childhood is unresponsive. In long-standing inflammatory and proliferative diseases, such as bronchiectasis or carcinoma, bronchial blood flow may be greatly increased. Scar tissue and tumors larger than 1 mm in diameter receive their blood supply via the bronchial circulation. The bronchial circulation is also the primary source of new vessels for repair of tissue after lung injury. As will be discussed near the end of this chapter, the bronchial circulation also supplies the visceral pleura of species that have thick visceral pleura, including humans.
Pulmonary Circulation
In humans the pulmonary artery enters each lung at the hilum in a loose connective tissue sheath adjacent to the main bronchus (see Fig. 1-1 ). The pulmonary artery travels adjacent to and branches with each airway generation down to the level of the respiratory bronchiole ( Fig. 1-17 ). The anatomic arrangements of the pulmonary arteries and the airways are a continual reminder of the relationship between perfusion and ventilation that determines the efficiency of normal lung function. Although the pulmonary veins also lie in loose connective tissue sheaths adjacent to the pulmonary artery and main-stem bronchus at the hilum, once inside the lung they follow Miller’s dictum that the veins will generally be found as far away from the arteries and airways as possible. Peripherally, in the respiratory tissue the pulmonary arteries branch out from the core of the terminal respiratory unit, whereas the veins occupy the surrounding connective tissue envelope ( Fig. 1-18 ). Each small muscular pulmonary artery supplies a specific volume of respiratory tissue, whereas the veins drain portions of several such zones.


Considerable quantitative data about the pulmonary circulation are available for the human lung ( Table 1-2 ). Although most of the intrapulmonary blood volume is in the larger vessels down to approximately 500 µm in diameter, nearly all of the surface area is in the smaller vessels. For example, the surface area of arterioles 13 to 500 µm in diameter exceeds that of the larger vessels by a factor of two, and the maximal capillary surface area is 20 times that of all other vessels.
Vessel Class (with Diameter) | Volume (mL) | Surface Area (m 2 ) | Reference No. |
---|---|---|---|
Arteries (>500 µm) | 68 | 0.4 | |
Arterioles (13–500 µm) | 18 | 1.0 | |
Capillaries (10 µm) | 60–200 | 50–70 | |
Venules (13–500 µm) | 13 | 1.2 | |
Veins (>500 µm) | 58 | 0.1 |
Because the vertical height of the lung at FRC is 24 cm (see Fig. 1-2 ), the pressure within the pulmonary blood vessels varies by 24 cm H 2 O over the full height of the lung. Thus, if pulmonary arterial pressure is taken as 20 cm H 2 O (15 mm Hg, 1.9 kPA) at the level of the main pulmonary artery, which is halfway up the height of the lung, pressure in the pulmonary arteries near the top of the lung will be 12 cm H 2 O, whereas pressure in pulmonary arteries near the bottom will be 36 cm H 2 O. Pulmonary venous pressure, which is 8 cm H 2 O at the level of the pulmonary artery in midchest (left atrial pressure), would be −4 cm H 2 O near the top of the lung and +20 cm H 2 O at the bottom. In the normal lung the blood volume is greater at the bottom because of increased luminal pressure, which expands those vessels and increases their volume. This effect of distention also decreases the contribution of the blood vessels at the bottom of the lung to total pulmonary vascular resistance.
From the time after birth through adulthood, the normal pulmonary circulation is a low-resistance circuit. The resistance is distributed somewhat differently, however, than in the systemic circulation, where the major drop in resistance is across the arterioles. Although the pressure drop along the pulmonary capillaries is only a few centimeters of water (similar to the pressure drop in systemic capillaries), the pulmonary arterial and venous resistances are low, so a relatively larger fraction of the total pulmonary vascular resistance (35% to 45%) resides in the alveolar capillaries at FRC. (For further information about pulmonary circulation in health and disease see Chapters 6 and 58 .)
Vasoactivity plays an important part in the local regulation of blood flow in relation to ventilation. Because smooth muscle can be found in the pulmonary vessels on both the arterial and the venous side down to precapillary and postcapillary vessels, any segment can contribute to active vasomotion. In pathologic conditions, vascular smooth muscle may extend down to the capillary level.
Theoretically, gas exchange may take place through the thin wall of almost any pulmonary vessel. At normal alveolar oxygen tensions, however, little oxygen and carbon dioxide is exchanged before the blood reaches the true capillaries. In the pulmonary arterioles, because of their small volume (see Table 1-2 ), blood flow is rapid. As blood enters the vast alveolar wall capillary network, its velocity slows, averaging approximately 1000 µm/sec (or 1 mm/sec). Flow in the microcirculation is pulsatile because of the low arterial resistance. Pulsations reach the microvascular bed from both the arterial and the venous sides. In fact, one sign of severe pulmonary hypertension is the disappearance of capillary pulsations.
The capillary network is long and crosses several alveoli ( Fig. 1-19 ) of the terminal respiratory unit before coalescing into venules. The vast extent of the capillary bed together with the length of the individual paths means a reasonable transit time for red blood cells, during which gas exchange can take place. The anatomic estimate of approximately 0.5 to 1 second average transit time is essentially the same as that found using the carbon monoxide diffusing capacity method, in which one divides capillary blood volume by cardiac output to obtain mean capillary transit time. In the normal lung sufficient time is available for equilibrium between the oxygen and carbon dioxide tensions in the alveoli and the erythrocytes in the pulmonary capillaries. Only under extreme stress (heavy exercise at low inspired oxygen tensions) or in severe restrictive lung disease would the red blood cells be predicted to pass through the microcirculation without enough time to reach diffusion equilibrium.

Normally, capillary blood volume is equal to or greater than stroke volume. Under normal resting conditions, the volume of blood in the pulmonary capillaries is well below its maximal capacity, however. Recruitment can increase this volume by a factor of about three. Thus the normal capillary blood volume of 60 to 75 mL is one third of the capacity (200 mL) measured by quantitative histologic analysis.
Anatomically, the pulmonary blood vessels can be divided into two groups in a manner similar to the connective tissue compartments: extra-alveolar and alveolar. Extra-alveolar vessels lie in the loose-binding connective tissue (peribronchovascular sheaths, interlobular septa). Extra-alveolar vessels extend into the terminal respiratory units. Arteries as small as 100 µm in diameter have loose connective tissue sheaths. This is in contrast to the bronchioles, which are tightly embedded in the lung framework from the bronchioles (1 mm in diameter) onward. Alveolar vessels lie within the alveolar walls and are embedded in the parenchymal connective tissue. They are subject to whatever forces operate at the alveolar level. They are referred to as alveolar vessels in the sense that the effective hydrostatic pressure external to them is alveolar pressure. Not all of the alveolar vessels are capillaries, however. Small arterioles and venules, which bulge into the air spaces, may be affected by changes in alveolar pressure. Likewise, not all of the capillary bed is alveolar under all conditions. The corner capillaries in the alveolar wall junctions are protected from the full effects of alveolar pressure by the curvature and alveolar air-liquid surface tension. This may account for the fact that, even under zone 1 conditions in which alveolar pressure exceeds both arterial and venous pressure, some blood continues to flow through the lung. One has to go several centimeters up into zone 1 before blood flow stops completely. (For a discussion of distribution of pulmonary blood flow and lung zones, see Chapter 4 .)
An important question is whether the normal human lung contains connections between the pulmonary arteries and veins that permit some portion of pulmonary blood flow to bypass the capillary network. Such vessels may develop congenitally or pathologically. In the normal lung, however, functioning short circuits probably do not exist. (Pathologic arteriovenous communications are discussed in Chapter 61 .)
Pulmonary capillaries are lined by continuous (nonfenestrated) endothelial cells ( Fig. 1-20 ). These attenuated cells have an individual area of 1000 to 3000 µm 2 and an average volume of 600 µm 3 . These large, flat cells cover a total surface area of approximately 130 m 2 . Other structural features of pulmonary capillary endothelial cells are the large number of plasmalemmal vesicles and small number of organelles (see Fig. 1-20 ). Despite having relatively few organelles, pulmonary capillary endothelial cells do have organelles involved in protein synthesis, such as endoplasmic reticulum, ribosomes and Golgi apparatus, and endocytosis (caveolae, multivesicular bodies, and lysosomes). The endocytic apparatus appears to participate in receptor-mediated uptake and transport (transcytosis) of albumin, low-density lipoproteins, and thyroxin. Another route for passage of solutes and water is between adjacent endothelial cells (transcellular transport). However, that passage route is restricted by specialized junctional complexes called “tight junctions.”

In addition to its function in gas exchange, the pulmonary circulation is involved in a number of other functions important to homeostasis. The pulmonary vascular bed serves as a capacitance reservoir between the right and left sides of the heart. Consequently, the reservoir of blood in the pulmonary circulation is sufficient to buffer changes in right ventricular output for two to three heartbeats. The pulmonary vascular bed also serves as a filter, trapping any embolic material from systemic vascular beds. For example, during intravascular coagulation or in processes involving platelet or neutrophil aggregation, the predominant site of sequestration is the lung. The main anatomic reason for this is that 75% of the total circulating blood volume is in the venous circuit, and the lung’s microvascular bed is the first set of small vessels through which the blood flows. Moderate numbers of microemboli generally produce no detectable dysfunction because of the huge array of parallel pathways in the microcirculation. At most, microemboli temporarily block flow to a portion of or to an entire terminal respiratory unit. The fate of such emboli is not clear. Some are phagocytosed and removed into the lung tissue. Some emboli can be degraded to a small size, pass through into the systemic circulation, and be removed by the reticuloendothelial system. One example of particulate matter that filters in the lung is the macroaggregated serum albumin used in lung-scanning procedures. (Further information about the pathophysiology of thromboembolic disorders is presented in Chapter 57 .)
The endothelial cells of the pulmonary circulation are capable of a remarkable number of metabolic activities. This is not to say that endothelial cells in other organs do not have similar activities. But the central position of the lung, through which the entire cardiac output passes, places extra responsibility and extra importance upon its endothelial cells. For example, angiotensin I, bradykinin, and prostaglandin E 1 are nearly completely inactivated during a single pass through the lungs. Pulmonary endothelial cells also express at least two subtypes of endothelin receptors (A and C). Their expression coincides with rapid removal of endothelin, suggesting that the lung microcirculation participates in clearance of this potent vasoconstrictor peptide from the blood. Conversely, a potent vasodilator, nitric oxide, is generated locally in the lung, through expression of endothelial nitric oxide synthase.
Endothelial cells may have a role in regulating vascular tone and reactivity. An indication of this regulatory role can be seen in the direct contacts between pulmonary endothelial cells in small arteries and veins and the surrounding smooth muscle cells. Such myoendothelial contacts have been described in the lungs of a number of small animals, and we have seen them in the human lung ( Fig. 1-21 ). Although their functional importance is unknown, they may have some bearing on endothelial-dependent vasoactivity.

Regulation of vasoactivity by endothelial cells may be facilitated by site-specific phenotypes of endothelial cells (reviewed by Garlanda and Dejana and Gebb and Stevens ). For example, endothelial nitric oxide protein is more evident in small pulmonary arterial vessels than in capillaries. Presumably the more evident localization reflects the functional role of nitric oxide in regulating pulmonary artery smooth muscle tone. On the other hand, capillary endothelial cells appear to have more expression-activated message for leukocyte adhesion molecules than arterial endothelial cells. Greater expression by capillary endothelial cells may contribute to sequestration of leukocytes in the capillary bed during acute inflammatory reactions. Another endothelial cell function that is site specific in the lung is Ca 2+ transients that are induced by pressure elevations as small as 5 cm H 2 O. The calcium transients seen in a subset of Ca 2+ oscillating cells are referred to as “pacemakers” and are located in pulmonary venular capillaries. The oscillations are propagated to adjacent endothelial cells. This endothelial response may be relevant in the pathogenesis of pressure-induced lung microvascular injury.
Terminal Respiratory Units
The “alveolus” of which the physician or pulmonary physiologist speaks is referred to as the “terminal respiratory unit” by the anatomist. The terminal respiratory unit consists of all the alveolar ducts, together with their accompanying alveoli, that stem from the most proximal (first) respiratory bronchiole (see Fig. 1-18 ). The terminal respiratory unit has both a structural and a functional existence and was first described by Hayek. In the human lung this unit contains approximately 100 alveolar ducts and 2000 alveoli. At FRC the unit is approximately 5 mm in diameter, with a volume of 0.02 mL. In normal adult humans, there are approximately 150,000 such units in both lungs combined. The acinus, an anatomic unit popular among pathologists, contains 10 to 12 terminal respiratory units.
The functional definition of the terminal respiratory unit is that, because gas phase diffusion is so rapid, the partial pressures of oxygen and carbon dioxide are uniform throughout the unit. Diffusion is the name for a thermodynamic process by which molecules express their kinetic energy. Net diffusion takes place when a concentration difference of a substance exists between two volumes. Thus oxygen in the alveolar duct gas will diffuse into the alveoli, because the incoming air has a higher oxygen concentration than the alveolar gas. Oxygen will also diffuse from the gas adjacent to the alveolar wall through the air-blood barrier into the red blood cells flowing in the capillaries ( Fig. 1-22 ), where oxygen combines with hemoglobin. Carbon dioxide diffuses in the opposite direction. A key point about diffusion is that the process is much faster in the gas phase than in water. Thus the terminal respiratory unit size is defined in part by the fact that gas molecules can diffuse and equilibrate anywhere within the unit more rapidly than they can diffuse through the membrane into the blood. The main problem is that the solubility of oxygen in water is low relative to its concentration in gas. Water becomes a problem when edema liquid accumulates in alveoli and/or interstitium in the alveolar walls. Carbon dioxide is much more soluble in water (20 times the solubility of oxygen in water), and therefore carbon dioxide diffuses rapidly into the gas phase, even though the driving pressure for carbon dioxide diffusion is only one tenth that for oxygen entering the blood.

It is almost impossible to demonstrate that diffusion is limiting in the normal lung, except during heavy exercise while breathing gas containing very low oxygen concentrations. Even then, diffusion limitation may not be as important as the reduced transit time of the red blood cells. However, apart from these observations during heavy exercise, most disorders of oxygenation are due to ventilation-perfusion inequalities.
All portions of the terminal respiratory unit participate in volume changes with breathing. Thus, if a unit were to increase its volume from FRC, the alveolar gas that had been in the alveolar duct system would enter the expanding alveoli, together with a small portion of the fresh air. Most of the fresh air would remain in the alveolar duct system. This does not lead to any significant gradient of alveolar oxygen and carbon dioxide partial pressures because diffusion in the gas phase is so rapid that equilibrium is established within a few milliseconds. But nondiffusible (suspended or particulate) matter would remain away from the alveolar walls and be expelled in the subsequent expiration. This explains why it is difficult to deposit aerosols on the alveolar walls and why large inspired volumes and breath-holding are important for obtaining efficient alveolar deposition.
The anatomic alveolus is not spherical ( Fig. 1-23 ; see Fig. 1-19 ). It is a complex geometric structure with flat walls and sharp curvature at the junctions between adjacent walls. The most stable configuration is for three alveolar walls to join together, as in foams. The resting volume of an alveolus is reached at minimal volume, which is 10% to 14% of total lung capacity. When alveoli go below their resting volume, they must fold up because their walls have a finite mass. Most of the work required to inflate the normal lung is expended across the air-liquid interface to overcome surface tension; the importance of the air-liquid interface is demonstrated by the low pressure required to “inflate” a liquid-filled lung with more liquid.

The phenomenon of terminal respiratory unit, or alveolar, stability is confused because not only is air-liquid interfacial tension involved, but each flat alveolar wall is part of two alveoli and both must participate in any change. Therefore atelectasis does not usually involve individual alveoli but rather relatively large units ( Fig. 1-24 ).

The alveolar walls are composed predominantly of pulmonary capillaries. In the congested alveolar wall, the blood volume may be more than 75% of the total wall volume. Alveoli near the top of the lung show less filling of the capillaries than those at the bottom. This affects regional diffusing capacity, which is dependent on the volume of red cells in the capillaries (see also eFig. 25-10 ).
The transition from the cuboidal epithelium of the respiratory bronchiole to alveolar squamous epithelium is abrupt (see Fig. 1-12 ). Although Macklin speculated that the permeability of the bronchiole-alveolar epithelial junctions may be special, no definitive difference has been demonstrated. The controversy continues as to whether this region shows unique permeability features that might participate in clearance of particles or leakage of edema.
The pleomorphic nature of the alveolar epithelium and the light and electron microscopic structure of its constituent cells have been described many times and will be only briefly summarized here. In normal mammals and other air-breathing species, including reptiles and amphibians, the alveolar epithelium is composed of cuboidal alveolar type II cells and flattened type I cells ( Fig. 1-25 ). Alveolar type II cells outnumber type I cells (≈15% versus 8% to 10% of total peripheral lung cells, respectively), but type I cells account for approximately 90% to 95% of the alveolar surface area of the peripheral lung. The two cell types have different functions and structure.
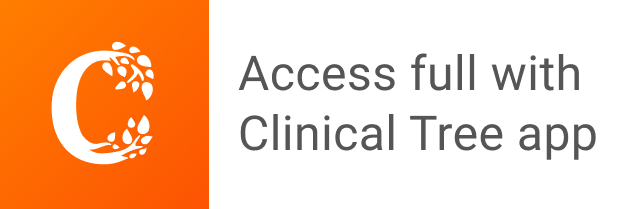