Introduction
The major function of the lung is to facilitate gas exchange between the air and blood compartments, which takes place in the alveolar region of the lung. In the adult lung, the alveolar-capillary barrier that permits efficient gas exchange is formed by thin cytoplasmic extensions of alveolar type I (TI) cells and capillary endothelial cells, separated by a common fused basement membrane.
The alveolar surface area comprises more than 99.5% of the large internal surface area of the lung, estimated in the adult human to be approximately 100 to 150 m 2 . Efficient diffusion of gases between the air and blood is dependent on the thin aqueous/lipid lining layer and the cellular compartments (epithelial, interstitial, and endothelial). Capillaries are located in the interalveolar septum that bridges different alveolar surfaces. On the air side, the alveolus is lined by two morphologically distinct epithelial cells, TI and alveolar type II (TII) cells. The morphologic and morphometric characteristics of both TI and TII cells are remarkably constant over an approximately 10,000-fold range in mammalian lung size, from which one may infer that there is a conservation of functions of both cell types. Although the distinctive anatomic features of alveolar cells permit the efficient diffusion of gases by forming a thin cellular barrier, alveolar epithelial cells have also evolved to perform other necessary functions, including producing pulmonary surfactant, maintaining an optimal alveolar liquid layer by regulating ion and water transport, protecting against inhaled toxins and infecting agents, and repairing the alveolar epithelium following lung injury or inflammation.
Pulmonary surface-active material (surfactant) is critical for normal lung function. Without surfactant, the work of breathing increases markedly and respiratory distress develops rapidly. In the absence of surfactant the work of breathing may increase from less than 2% to more than 10% of total oxygen consumption. Surfactant provides the low surface tension at the air-liquid interface that is necessary to prevent atelectasis, alveolar flooding, and severe hypoxia. A complex but highly regulated process has evolved for the synthesis, secretion, and reutilization of surface-active material. In addition to its well-recognized property of providing alveolar stability, surfactant is also important for maintaining the patency of small airways and preventing alveolar flooding.
This chapter reviews the functions of TI cells and TII cells, the physiologic properties of pulmonary surfactant, the use of surfactant replacement therapy in the treatment of hypoxic respiratory failure ( newborn respiratory distress syndrome [NRDS]), and the role of the alveolar epithelium and surfactant in selected lung diseases. Additional discussion of the alveolar epithelium can be found in Chapter 1 , and comprehensive reviews of acute respiratory distress syndrome (ARDS) can be found in Chapter 100 .
Alveoli
The number of alveoli in a lung is largely dependent on the size of the lungs, with larger lungs containing more alveoli. On average, Ochs and colleagues have estimated that the adult human lung contains approximately 480 million alveoli, each approximately 4.2 × 10 6 cu µ in size. Recently, the number of the alveoli in the mouse lung has been estimated to be 560 in young mice (12 weeks) and 880 in old mice (91 weeks); these estimates were derived by use of computed tomography imaging, a technique that is quite different from traditional stereology, and may allow quantitative measurements in disease and lung injury models. In the adult lung, the average alveolar fluid depth is estimated to be 0.14 to 0.20 µm.
Alveolar Epithelial Intercellular Junctions
Early physiologic studies demonstrated that transport of water and low-molecular-weight solutes out of the pulmonary capillaries is rapid, whereas transport of solutes out of the alveoli is extremely slow. The anatomic basis for the barrier function of the alveolar epithelium became clear from freeze-fracture studies of the lung that demonstrated that epithelial intercellular junctions had morphologic characteristics of “tight junctions,” in contrast to endothelial intercellular junctions that had morphologic characteristics of gap or “leaky” junctions.
Epithelial cells are attached to one another at their lateral membranes by a series of different junctional complexes, including tight junctions, adherens junctions, and gap junctions. These junctional complexes are each composed of different proteins that convey specific functions. Although both tight junctions and adherens junctions associate with the actin cytoskeleton, they have somewhat different functions; tight junctions regulate paracellular movement of ions and solutes, whereas adherens junctions mediate cell-cell adhesion and participate in cell signaling.
The most apical of the intercellular junctions is the tight junction or zona occludens (e.g., “closing belt”). By ultrastructural analyses, tight junctions appear as a series of anatomic appositions between the lateral membranes of adjacent cells that extend in a beltlike network surrounding cells, attaching them to adjacent cells and forming a continuous seal. The tight junction is the major factor in determining paracellular permeability. In addition to the “barrier” functions that regulate the passage of water, ion, and various molecules through paracellular spaces, tight junctions also have a “fence” function, which prevents intermixing of proteins and lipids in the apical and lateral membranes, establishing cell polarity. The tight junction is composed of a dynamic complex of proteins that include transmembrane proteins, cytoplasmic scaffolding proteins, signaling proteins, and adaptors that link to the cytoskeleton. Claudins play key roles in forming tight junction strands and determining paracellular permeability. Permeability can be further modulated by various other factors, including Rho-associated protein kinase, protein kinase C, and other kinases, as well as various cytokines, growth factors, and hormones.
A second type of intercellular junction, the adherens junction, is located more basally. Adherens junctions are composed of clusters of adherens molecules, such as vascular endothelial-cadherin, β-catenin, and plakoglobin, linked to the actin cytoskeleton. There are also associations with kinases, phosphatases, and growth factor receptors. Complex intermolecular interactions mediate cell-cell adhesion and modulate cell signaling from growth factors and from mechanical forces such as sheer stress. Adherens junctions play important roles in both development and wound healing. The complex field of adherences junctions has been well reviewed.
A third type of junction, the gap junction, is ubiquitous in most mammalian tissues. Gap junctions are composed of connexins, proteins that form intercellular channels, enabling the diffusion of molecules throughout interconnected cells, enabling cells to function in a coordinated measure. In the alveolar epithelium, gap junctions mediate intercellular calcium fluxes, which play a role in surfactant secretion.
Alveolar Type I Cells
In the mammalian lung, TI cells comprise only approximately 10% of the cells in the alveolar region but cover more than 95% of the internal alveolar surface area; TII cells (≈18% of alveolar cells) cover the remaining less than 5% of the surface area. TI cells are large (surface area ≈7 × 10 3 sq µm) squamous cells with thin cytoplasmic extensions that form the epithelial component of the air-blood barrier. The cytoplasmic extensions of TI cells are only 50 to 100 nm thick, below the resolution of conventional light microscopy. Prior to the development of the electron microscope, there was intense debate about whether the lining of the lung was cellular or acellular. Electron microscopic studies definitively demonstrated that the alveolar surface was lined by a continuous epithelium. TII cells are much smaller cells (≈30% of the volume and 3% of the surface area of TI cells) that are often, but not always, cuboidal in shape ( Fig. 8-1 ). Both TI cells and TII cells are not necessarily confined to one side of the alveolar septum but can bridge two or three different alveoli (see Fig. 8-3B ). These complex three-dimensional morphologic relationships may complicate simple interpretations of cellular function that assume distinct apical, basal, and lateral membrane domains.

We have learned a considerable amount about the biology of TII cells from the study of isolated and cultured TII cells and the development of transgenic mouse models. In contrast, techniques to isolate and study TI cells took many years to develop because of the lack of biochemical or molecular reagents to isolate and evaluate the cells and the fragility of isolated TI cells. Until fairly recently, the accepted concept was that the TI cell was a biologically inactive, terminally differentiated cell. However, recent evidence based on studies of isolated TI cells and gene expression profiling suggests that TI cells play important roles in numerous alveolar functions.
Many published images show only small portions of TI cells because of the large surface area of the cell and limited sampling area of electron microscopy. These images have given rise to an incorrect impression that TI cells do not contain either microvilli or subcellular organelles necessary for biosynthetic and other pathways. In fact, TI cells contain microvilli, abundant mitochondria, Golgi, rough and smooth endoplasmic reticulum, small intracellular vesicles, and caveolae, subcellular domains consistent with both metabolic and endocytic functions ( Fig. 8-2 ).





More detailed analyses of the differences in TI cells and TII cells from both specific studies and gene expression profiling have provided some insight into the functional differences between the two cell types. For example, there are differences in the expression of intercellular junctional proteins. Both TI and TII cells express primarily three claudins (claudin-3, claudin-4, and claudin-18.1). Claudin-3 is associated with decreased barrier function, whereas claudins 4 and 18 are associated with increased barrier function. The proportion of each claudin is different between the two cell types. In TI cells, the lung-specific claudin-18.1 constitutes more than 50% of claudin mRNA, whereas in TII cells, claudin-3 is the dominant claudin. Because TII cells contain approximately 20-fold more claudin-3 protein than do TI cells, it has been suggested that claudin-3 is localized mainly to TI-TII cell junctions and that TI-TII and TI-TI cell junctions may have different paracellular permeability characteristics; for example, it has been proposed that TI-TII cell junctions are more permeable than the TI-TI cell junctions that constitute the majority of the alveolar epithelial surface. There may be other differences between TI-TI and TI-TII junctions. For example, in models of lung injury, neutrophils appear to migrate across the epithelial barrier selectively between TI and TII cells, although the molecular mechanisms responsible for this apparent selectivity are unknown.
Our concepts about alveolar fluid homeostasis have evolved over the past 10 years. Studies of clearance of exogenously added lung liquid demonstrate rapid clearance of liquid from the alveolar space, suggesting that clearance is mediated by Na + channels with a high conductance and/or large numbers of channels with a lower conductance. Ions are transported actively, with water following passively. The osmotic water permeability of TI cells is one of the highest found in mammalian cell membranes, suggesting that TI cells play a major role in passive water transport. Presumably aquaporin 5, which is localized in the apical plasma membrane of TI cells, is responsible for the high osmotic water permeability; the molecular basis for water transport across the basal surface remains unknown. TI and TII cells also differ in the content of certain ion channels. Studies with either freshly isolated TI and TII cells or TII cells cultured under special conditions demonstrated that both cell types contain high-conductance sodium channels. TI cells, unlike TII cells, contain cyclic nucleotide gated (CNG) channels and K + channels, and TII cells contain more cystic fibrosis transmembrane conductance regulator (CFTR) than do TI cells per unit area. By immunohistochemical methods and Western blotting, TI cells contain the epithelial sodium channel (ENaC) and various subunits of the Na + -, K + -ATPase. In addition to high-conductance sodium channels, both cell types contain less selective Na+ channels, although these are far less abundant. In most species, less than half of fluid transport in the lung is inhibitable by amiloride ; thus, it has been postulated that the amiloride-insensitive CNG channels are responsible for the bulk of transport. The importance of amiloride-insensitive sodium transport was recently reviewed by O’Brodovich and associates. Although patch clamping data are consistent with the presence of CNG channels in TI cells but not TII cells, the presence of CNG channels in TII cells cannot be definitively excluded.
Whereas, in the adult, the epithelium from the alveolus to the nose is, in general, a sodium absorbing epithelium, in utero the alveolar epithelium actively secretes chloride into the developing airspace. Chloride secretion in the adult alveolar epithelium has not been clearly established and probably varies depending on microenvironmental conditions. However, human alveolar epithelial cells in vitro can be stimulated to secrete chloride. Both TI cells (CFTR, Cl − / anion exchanger, and voltage-gated Cl − channels) and TII cells (CFTR) contain Cl − channels, and TI cells have been shown to transport Cl − . In addition, the TII cell has a proton sodium exchanger that is likely important in acidifying alveolar fluid.
The pump linked to active sodium resorption is the Na + -, K + -ATPase. It has been estimated that approximately 60% of unstimulated fluid transport in the lung is mediated through the α2 subunit of Na + -, K + -ATPase, which is expressed in TI cells but not in TII cells. Data at present support the hypothesis that ions and water are transported across the entire alveolar epithelium. Taken together, the large discrepancy in surface area between the cell types, the channel density per unit area ( Table 8-1 ), the likely central role for CNG channels, and the relative importance of the α2 subunit of Na + -, K + -ATPase all suggest that the TI cell, rather than the TII cell, plays the major role in bulk fluid transport in the alveolar region.
TI : TII Cell Ratio | References | |
---|---|---|
Cell surface area | 43 | |
Osmotic water permeability | 7 | |
Na + and K + uptake/µg protein | ≈3 | |
Apical Na + channels/cell | ≈40 | |
CNG and K + channels | * | |
CFTR | 6 |
The innate immune functions of TII cells have been well documented, but it is only recently that TI cells have also been shown to have potential immunomodulatory functions. TI cells produce proinflammatory cytokines such as TNF-α, IL-6, and IL-1β after treatment with lipopolysaccharide (LPS). The production of cytokines in TI cells appears to be modulated by the renin-angiotensin system.
TI cells appear to be more susceptible to acute lung injury than are TII cells, and their cellular repair processes are relevant to restoring lung function after injury. TI cells contain abundant caveolae (see Fig. 8-2D ) and express caveolin-1. Caveolae have diverse functions including endocytosis and membrane trafficking, and, in addition, they are believed to play an important protective role by providing a source of membrane to protect against cellular lysis. Recent studies of TI cells injured in vitro have confirmed that membrane repair by lipid recruitment is facilitated by the caveolar endocytic pathway and by the remodeling of the actin cytoskeleton close to the site of wounding.
On the basis of studies in animal models of lung injury, it has been believed that the injured alveolar epithelium is repaired by proliferation and transdifferentiation of TII cells to TI cells, with TI cells regarded as terminally differentiated cells without proliferative potential. However, on the basis of recent in vitro studies, cultured TI cells have been shown to have a high proliferative potential. Both the potential proliferative capacity and phenotypic plasticity of the TI cell in vitro raise the possibility that TI cells in vivo may participate in lung repair after injury.
Some researchers have stressed the concept that pulmonary epithelium in the conducting airways can sense the environment (e.g., oxidant gases, cigarette smoke, nanoparticles) (see Chapter 10 ). If this is also true in the alveolus, then it likely that the TI cells, which cover nearly all the alveolar surface, will be shown to be important sensors of the environment.
Alveolar Type II Cells
TII cells were first comprehensively described by C. C. Macklin before the advent of electron microscopy. Many of his initial predictions about the function of these cells have been verified over the past 60 years. In electron micrographs, TII cells are readily identified by the presence of lamellar bodies, the intracellular storage form of pulmonary surfactant ( Fig. 8-3 ). The major functions of TII cells include: producing pulmonary surfactant, serving as the progenitor cells for maintaining the alveolar epithelium under normal circumstances and after mild injury, transporting fluid to keep the alveoli from flooding, and playing an important role in innate immunity. The high density of mitochondria and high glucose and oxygen consumption indicate that TII cells are highly metabolic. Because TII cells are the only source of surfactant, which is rich in phospholipids, lipid metabolism of the TII cells has been studied extensively. TII cells are highly enriched in genes involved in lipid synthesis. For example, TII cells can be identified in the lung by expression of two enzymes of lipid metabolism, fatty acid synthase and stearoyl CoA desaturase-1, as well as by expression of the traditional markers such as surfactant protein (SP) C. Lipogenesis is upregulated at the end of gestation and is regulated by the transcription factors sterol regulatory element binding protein-1c and CCAAT/enhancer binding protein (C/EBP) alpha, similar to other lipogenic cells. TII cells also are unique in expression of one of the surfactant proteins, SP-C, and the promoter for SP-C has been extremely useful for expressing or deleting genes in murine TII cells. However, while the TII cells express other surfactant proteins, SP-A, SP-B, and SP-D, these proteins are also expressed in nonciliated bronchiolar cells (club cells [Clara]) and at low levels in some extrapulmonary issues.

The turnover of TII cells in the normal, uninflamed lung is relatively slow. However, in response to injury to TI cells, TII cells proliferate rapidly to restore the epithelium. These TII cells become a transit-amplifying population. The initial studies to demonstrate this relationship used oxidant injuries in rodents to identify the proliferating cells with titrated thymidine. In these studies, there was an initial labeling of TII cells and, with time, the labeled cells differentiated into TI cells. More recently, the ability of TII cells to proliferate and transdifferentiate into TI cells was documented by lineage tracing techniques in mouse lung. This recent report demonstrated that TII cells are the source of new TI cells and TII cells under normal conditions and under conditions of mild injury. Hence, TII cells can serve as a transient proliferating cell population to maintain the alveolar epithelium under normal circumstances. However, in response to severe injury such as after influenza or toxins specific to TII cells, other epithelial cells from the bronchioalveolar junction or terminal airways that express keratin V, α 6 /β 4 integrin, or club cell secretory protein (Clara), can proliferate, migrate, and, in some circumstances, express SP-C. These additional pathways have been reported in mice and, although they are not fully defined, may be important in ARDS. Although the precise regulation of the transition of the TII cell to TI cell phenotype is not known, bone morphogenic protein 2 and transforming growth factor-β 1 (TGF-β1) have been suggested as regulators of the TII cell to TI cell transdifferentiation in vitro. Keratinocyte growth factor and hepatocyte growth factor are also important mitogens for TII cells in vivo and in vitro.
As stated earlier, alveolar TII cells can also transport sodium and chloride to help maintain alveolar fluid volume. This apical to basal surface fluid transport was first shown in vitro by the formation of domes, collections of fluid under the cells that lifted them up into a domelike structure. Under normal circumstances, TII cells resorb fluid by transporting sodium through amiloride-sensitive and amiloride-insensitive sodium channels, thereby keeping the alveolus relatively free of fluid. However, under certain in vitro conditions, TII cells can also secrete chloride. Unfortunately, defining the precise transport functions of alveolar TII cells in vitro is compromised by the fact that the culture conditions employed to study transport do not maintain the TII cell phenotype, as determined by the loss of expression of cell differentiation–specific markers, such as the surfactant proteins.
Alveolar TII cells are also important in the initial innate immune response to environmental insults, such as air pollutants, toxins, bacteria, and viruses. Alveolar TII cells are part of the first responders when microbes and other toxic agents enter the alveolus. Surfactant proteins A and D (SP-A and SP-D) are important components of the innate immune system and are discussed in detail later in this chapter. SP-A and SP-D are multivalent lectins (i.e., proteins that bind to carbohydrates and can play numerous roles in biologic recognition). Indeed, SP-A and SP-D can bind to a variety of viruses, bacteria, and fungi. However, TII cells can also be the target of specific respiratory viruses such as SARS-CoV and influenza. For example, if influenza is instilled into excised human lung, it mainly infects TII cells and does not infect TI cells. In response to viral infection, TII cells secrete a variety of cytokines to activate macrophages, recruit monocytes, and trigger the adaptive immune system. The dominant interferon produced by TII cells is interferon-λ (IL29), a type III interferon. The overall magnitude of the cytokine response is robust and similar to the response in alveolar macrophages. The major cytokines produced in response to influenza include CXCL10, IL6, RANTES, and IL29. Microbes can be recognized by pathogen recognition receptors (PRRs), such as Toll-like receptors (TLRs). At the mRNA level, human TII cells have significant expression of TLR2, TLR3, and TLR5 but low expression of TLR4 and TLR7, similar to that in bronchial epithelial cells. TII cells can also respond to danger-associated molecular patterns (DAMPs).
TII cells are thought to be important in various lung diseases. In ARDS and models of acute lung injury, TII cells can repair the alveolar epithelium, although it is possible that other cell populations also participate in this process during severe injury. TII cells are necessary for restoring the surfactant system, which is critical for gas exchange and for minimizing transudation of fluid into the alveolar space. In interstitial lung disease, TII cell hyperplasia is a common pathologic feature. One of the current hypotheses on the pathogenesis of idiopathic pulmonary fibrosis (IPF) is that the fibrotic response is caused by TII cell dysfunction due to protein misfolding and endoplasmic reticulum (ER) stress, which ultimately leads to secretion of TGF-β and other profibrogenic factors. Although some have considered an epithelial mesenchymal transition (EMT) to be an important part of the fibrotic response, this is unlikely to be important on the basis of recent lineage tracing studies. Mutations of SP-A and SP-C are thought to cause some types of familial pulmonary fibrosis, and this is thought to be due to protein misfolding and endoplasmic reticular stress. Potentially more will be learned about the role of TII cells in interstitial lung disease when these cells are isolated from diseased lung. Finally, TII cells can be a source of adenocarcinomas. Bronchioalveolar carcinomas and some adenocarcinomas likely arise in TII cells. Surfactant proteins and the transcription factor TTF1 have been used to differentiate lung adenocarcinomas from extrapulmonary sources and the presence of micrometastases in regional lung nodes in staging adenocarcinomas. In the mouse, urethane adenomas all express SP-C, which indicates that they are of TII cell origin and adenocarcinomas induced by KRAS mutations are also of TII cell origin.
Physiologic Functions of Pulmonary Surfactant
The discovery of pulmonary surfactant came from direct physiologic observation and an understanding of the Laplace relationship for calculating surface tension. In 1929, von Neergaard discovered that there was a difference in the elastic recoil properties of the lung depending on whether the lung was inflated with air or saline so that it took more pressure to inflate a lung with air than with saline ( Fig. 8-4 ). Even though it takes more pressure to inflate the lungs with air than with saline, von Neergaard calculated that the pressure needed to inflate the lungs with air was much less than expected at low lung volumes. From these observations, von Neergaard deduced that the surface tension in the lung was low. By studying extracts of lung, Clements and Pattle independently demonstrated that surfactant, specifically the phospholipids of surfactant, was responsible for lowering the surface tension. The low surface tension, produced by pulmonary surfactant, markedly decreases the work of breathing, prevents alveolar collapse and atelectasis, allows for alveoli of different size (radius of curvature) to be stable, and prevents alveolar flooding. Soon after these discoveries, Avery and Mead demonstrated that a deficiency in surface-active material caused NRDS. The critical component of surfactant that provides the low surface tension is dipalmitoylphosphatidylcholine (DPPC). This is an unusual species of phosphatidylcholine in that both fatty acids are saturated. DPPC molecules can pack closely together and allow the surface monolayer to withstand the high film pressures required to produce a low surface tension at low lung volumes.

Film pressure is the pressure required to maintain a surface film and is easiest measured and conceptualized in a Langmuir-Whilhemy balance in which a moveable barrier is used to compress a surface monolayer. Compression of the surface balance reduces the area of the surface film similar to exhalation. Surfactant produces a stable film and is able to withstand high film pressures without collapsing. During inflation, surfactant must be absorbed into the surface. During deflation, as the surface area decreases, the film pressure rises and the surface tension falls. Phospholipid molecules already present in the air-liquid interface get packed closely together. High film pressures squeeze some components of the film, such as unsaturated phospholipids and the surfactant proteins, out of the monolayer. The surface film generated at low lung volumes is thought to be composed of nearly pure (95%) DPPC. Epifluorescence studies with hydrophilic markers have shown that, as the film is compressed, there are areas from which aqueous markers are excluded. Fixation of the lung with osmium vapors has shown that some of the alveolar surface is covered with multilayers of phospholipids, which serve as a reservoir of surfactant to enter the film at high lung volumes. The surfactant system provides a low surface tension in the alveoli and small airways but not in the large airways or trachea.
There are four surfactant-associated proteins, each of which has different functions. From in vitro studies on film formation, it is clear that SP-A, SP-B, and SP-C can increase the rate of delivery of phospholipids to the air-liquid interface; by itself, DPPC has an extremely low rate of adsorption to the surface. In addition, SP-A and SP-B were found to be necessary for the formation of tubular myelin ( Fig. 8-5 ). Tubular myelin is a unique extracellular form of surfactant, in which an organized lattice of surfactant bilayers is formed. These bilayers appear to form at right angles in both transmission electron micrographs and freeze fracture images. Tubular myelin is the form of surfactant intermediate between the lamellar body, which is secreted, and the surface monolayer. Tubular myelin is a major component of large-aggregate surfactant, the form that sediments rapidly on centrifugation and is surface active. However, because SP-A–deficient mice lack tubular myelin and have normal respiratory mechanics, the formation of tubular myelin is not absolutely necessary for surfactant function.

The major physiologic function of surfactant is to lower surface tension and thereby provide alveolar stability, but surfactant has other functions as well. Surfactant is important for maintaining the patency and stabilization of small airways. Studies in narrow fluid-filled tubes have demonstrated the critical importance of low surface tension and the need for added surfactant to reduce opening pressures. For this reason, surfactant is important in asthma, constrictive bronchiolitis, and other diseases of small airways. Finally, there is compelling evidence that SP-A and SP-D play important roles in host defense (see later).
Composition and Pool Sizes
The critical components of purified surfactant are DPPC, unsaturated phosphatidylcholine, phosphatidylglycerol, and the surfactant proteins ( Table 8-2 ). In a variety of species, the quantity of saturated phosphatidylcholine is related to the alveolar surface area. Phosphatidylglycerol is an anionic phospholipid that is thought to be important in the electrostatic and calcium-dependent interactions with the surfactant proteins. In lung injury, a reduction in the percentage of phosphatidylglycerol is the earliest and most sensitive alteration in the composition of surfactant. Recently, pure phosphatidylglycerol liposomes have been reported to have antiviral properties. However, the phosphatidylglycerol found in mixed micelles with other phospholipids in natural surfactant likely does not have significant antiviral properties. The mechanism by which pool sizes of surfactant are regulated is not understood. However, recently it has been suggested that the TII cell senses the pool of surfactant via SP-D and the orphan receptor Hepta/GPR116. A major physiologic regulator of surfactant pool size is clearance by alveolar macrophages as discussed later in this chapter and in Chapter 70 .
PHOSPHOLIPIDS: 85% * | % OF PHOSPHOLIPIDS |
Phosphatidylcholine | 76.3 |
Dipalmitoylphosphatidylcholine | 47.0 |
Unsaturated phosphatidylcholine | 29.3 |
Phosphatidylglycerol | 11.6 |
Phosphatidylinositol | 3.9 |
Phosphatidylethanolamine | 3.3 |
Sphingomyelin | 1.5 |
Other | 3.4 |
NEUTRAL LIPIDS: 5% † | |
Cholesterol, free fatty acids | |
PROTEINS: 10% ‡ | |
SP-A | ++++ |
SP-B | + |
SP-C | + |
SP-D | ++ |
Other |
* The phospholipid composition is constant in most mammalian species. Disaturated phosphatidylcholine represents about two thirds of the total phosphatidylcholine. Dipalmitoylphosphatidylcholine makes up the majority species of the disaturated phosphatidylcholine fraction and is the critical molecule for providing the low surface tension.
† There is about 5% neutral lipid, most of which is cholesterol and free fatty acids. There is relatively little triglyceride and cholesterol ester.
‡ The composition of the surfactant proteins is not known precisely, but on a mass basis there appears to be more SP-A than SP-D and more SP-A than SP-B and SP-C. However, there is significant uncertainty about the exact values for SP-B and SP-C.
Surfactant Protein A
SP-A was the first surfactant protein identified by its association with surfactant phospholipids ( Fig. 8-6 ). SP-A is a large octadecameric protein with a molecular mass of about 650 kDa. SP-A is a collagenous glycoprotein with a complex, highly ordered tertiary structure. The overall organizational structure of SP-A is similar to serum mannose-binding lectin and the complement component C1q. These molecules form a polarized bouquet-like structure composed of 18 monomers that are organized as six trimeric units. The C-terminal carbohydrate recognition domain (CRD) is critical for most SP-A functions and consists of a globular domain that binds carbohydrate and other ligands recognized by SP-A. The structure of the CRD is highly conserved in this class of calcium-dependent lectins. The macromolecular structure of SP-A is 20 nm from the N-terminal to the C-terminal CRD unit and across the array of CRD units. The crystal structure has revealed a hydrophobic binding pocket in the CRD that likely accounts for the lipid binding properties of SP-A.

SP-A is localized primarily in the gas-exchange units of the lung and small airways. In rodents, SP-A is found in alveolar TII cells and also in nonciliated bronchiolar cells (club cells) that line the conducting airways. In humans almost all the SP-A is found in the alveoli, and little is found in the respiratory pseudostratified epithelium that lines the conducting airways. However, there is SP-A in human tracheal submucosal glands.
The gene for SP-A is located on chromosome 10 near the closely related proteins, SP-D and mannose-binding lectin. Humans have two genes for SP-A, which code for proteins with minor amino acid alterations in the collagen domain. Newly synthesized SP-A undergoes a variety of posttranslational modifications that include proteolytic removal of the signal peptide, addition of N-linked carbohydrates, sialylation, acetylation, and sulfation. Secreted SP-A is highly glycosylated, whereas most of the intracellular form of SP-A is not. A number of factors have been reported to increase the synthesis of SP-A, including cyclic adenosine monophosphate (AMP), keratinocyte growth factor, and interleukin (IL)-1. Corticosteroids produce a biphasic dose response with stimulation at low concentrations and inhibition at high concentrations.
SP-A is secreted from TII cells by two different routes. The dominant route is by direct constitutive secretion independent of exocytosis of the lamellar bodies. In addition, there is secretion of SP-A contained within lamellar bodies. Directly secreted SP-A is newly synthesized, whereas the SP-A found in lamellar bodies is likely derived from recycled SP-A.
In vitro the functions of SP-A include lipid binding and formation of tubular myelin, acceleration of the adsorption of surfactant to the air-liquid interface, and inhibition of surfactant secretion. However, the physiologic importance of these in vitro observations on surfactant regulation has been seriously questioned because the SP-A–deficient mouse has normal surfactant function. More than 99% of SP-A in lavage fluid is bound to phospholipid. SP-A may also be important for maintaining the function of surfactant during acute lung injury, when a variety of serum factors can inhibit surfactant function; SP-A partially inhibits these effects.
However, the major function of SP-A appears to be in innate immunity; SP-A binds to a variety of microorganisms, promotes their clearance by phagocytic cells, and directly alters the function of immune effector cells. SP-A, like SP-D, is a multivalent pattern recognition molecule and binds to a wide variety of glycoproteins and other ligands. Because the binding is somewhat promiscuous with a low affinity, the physiologic effects of SP-A are likely due to its polyvalent structure and multiple binding sites on cells and organisms. It has been proposed that SP-A associated with tubular myelin might be one of the first barriers for host protection; multivalent SP-A could bind both surfactant lipid in alveolar fluid and inhaled organisms or particles.
SP-A binds to both gram-positive and gram-negative bacteria and is thought to be an important component of host defense. SP-A binds gram-negative bacteria with the rough form of LPS, aggregates these bacteria, and increases phagocytosis and killing. SP-A binds poorly to the smooth variants of Escherichia coli . Gram-negative bacteria that colonize the respiratory tract usually display the smooth form of LPS, whereas those that colonize the gastrointestinal tract display rough forms of LPS. SP-A also enhances the adherence and subsequent phagocytosis of mycobacteria by macrophages. Lipoglycans, especially mannosylated lipoarabinomannan, are important ligands for SP-A on mycobacteria. SP-A also binds to a variety of viruses including influenza and respiratory syncytial virus and likely aggregates extracellular virus, which may inhibit infection.
SP-A has been reported to bind to several receptors on macrophages and to modulate the expression of microcidal factors, TLR2 and 4, and the clearance of apoptotic cells. The binding of SP-A to alveolar macrophages appears to be specific and not seen to other mononuclear phagocytes as Kupffer cells, resident peritoneal macrophages, or peritoneal macrophages. The studies on direct activation of inflammatory cells are complex and depend on the method of SP-A isolation and the state of the inflammatory cells, especially if they have been primed by a cytokine such as interferon-γ. In addition, for in vitro studies to simulate the in vivo situation, it is important that the effect of SP-A is assessed in the presence of surfactant phospholipids because SP-A may bind surfactant phospholipids with a higher affinity than microbes or inflammatory cells. Finally, some preparations of SP-A may have contained TGF-β, which could account for some anti-inflammatory properties.
SP-A appears to suppress the secretion of inflammatory cytokines by macrophages in the normal lung but enhances cytokine production during infection or lung injury. This is sometimes referred to as the inflammatory paradox of SP-A. Gardai and colleagues formulated an interesting mechanism for these observations. In the normal setting, SP-A interacts with macrophages via its CRD domain and binds to signal-inhibitory regulatory protein α, which suppresses cytokine production. However, during infection, SP-A binds the invading organism with its CRD domain and instead interacts with macrophages via its N-terminal domain and binds to the calreticulin/CD91 complex to stimulate the production of inflammatory cytokines. Hence, SP-A can both stimulate and inhibit inflammatory cytokine production in vitro. It is important to recall that in vivo there is a complex interaction among SP-A, the phospholipids of surfactant, inhaled microbes, and receptors on inflammatory cells. Each has a different binding affinity for SP-A and independent regulation. Finally, SP-A has also been shown to bind to apoptotic cells and increase their uptake and removal by macrophages, although SP-A appears to be less important than SP-D in the clearance of apoptotic cells in vivo (see Chapter 12 ).
Identifying functional cell receptors for SP-A and SP-D has been challenging because both are polyvalent lectins and can bind to a variety of glycoproteins and glycolipids. Receptors for SP-A are presumably on TII cells for surfactant recycling and on macrophages for surfactant clearance and modulation of the innate immune response. There have been several putative receptors on macrophages, including the calreticulin/CD91 complex, signal-inhibitory regulatory protein α, and SP-R210. The major functional receptor for SP-A on TII cells is P63 (CKAP4). Currently, we do not know the precise role of these receptors in normal surfactant metabolism or in disease.
SP-A also serves as a fetal hormone of parturition. Mendelson and colleagues have suggested that the fetal lung secretes SP-A, which activates fetal macrophages that migrate to the uterine wall, where they produce IL-1β and initiate labor.
SP-A–deficient mice have demonstrated the importance of SP-A in host defense for viral and bacterial pathogens. SP-A–deficient mice are more susceptible to infection by a variety of organisms. There are no reported genetic diseases due to the deficiency of SP-A in humans. However, there is a suggestion that mutation in SP-A may contribute to a familial form of pulmonary fibrosis.
In summary, SP-A is bound to pulmonary surfactant phospholipids in vivo and functions primarily in innate immunity and host defense.
Surfactant Protein B
SP-B is the only surfactant protein that has been demonstrated to be crucial for surfactant function and is thought to be critical for the adsorption and surface spreading of phospholipids. Mature SP-B is a homodimer composed of two 79 amino acid polypeptide chains linked by disulfide bonds. The monomer has an expected mass of 8.7 kDa and the homodimer 17.4 kDa. Each monomer has five amphipathic helices that interact with the surface of the monolayer but do not span the monolayer. There are three internal disulfide bridges within the monomer (C8–C17, C11–C71, and C35–C46) and an interchain disulfide at C48.
There is one gene for SP-B that is located on human chromosome 2. The synthesis of SP-B is markedly stimulated by corticosteroids in vitro. This protein undergoes extensive intracellular processing before the mature homodimer is formed. Within the ER, the signal peptide is cleaved and a precursor 42-kd species of SP-B is formed. In multivesicular bodies, additional proteolysis removes a 16-kd amino terminal peptide and a 30-kd carboxy terminal peptide. The final processing of SP-B probably takes place within the multivesicular bodies, and the mature SP-B homodimer is formed by the time it arrives in the lamellar body.
SP-B is required for organizing phospholipids in lamellar bodies, for the formation of tubular myelin, and for delivering phospholipids to the alveolar air-liquid interface. SP-B is thought to be important in film formation and in the entry of phospholipids into the surface monolayer when the film is expanded during inspiration. The five amphipathic helices of SP-B are envisioned to lie along the lipid bilayer or surface monolayer. SP-B interacts with phospholipid bilayers by electrostatic interactions between the polar head groups of the phospholipids and its positively charged amino acids and through the nonpolar faces of the amphipathic helices that interact with the phospholipid acyl chains. SP-B is positively charged and has a preference for interacting with negatively charged phospholipids, such as phosphatidylglycerol. This surfactant protein is squeezed out of the monolayer at moderate film pressures (40 to 45 mN/m) and does not insert directly into the monolayer or lipid bilayers. SP-B can convert lipid vesicles into phospholipid sheets and is likely to be important in moving lipid into the monolayer with each respiratory cycle.
Mice with homozygous null alleles for SP-B die of respiratory insufficiency shortly after birth, and heterozygotes have impaired surfactant function. In genetically targeted mice with conditional expression of SP-B, reduction of SP-B below 25% of the wild-type level results in respiratory failure. As demonstrated in congenital SP-B deficiency in infants and in genetically altered mice, SP-B is absolutely required for the formation of highly structured lamellar bodies. SP-B is secreted exclusively as a component of lamellar bodies, and lamellar body formation may depend on the ability of SP-B to organize and bind two adjacent lipid bilayers. SP-B is found in natural surfactant preparations, although the content may vary from batch to batch and may differ from that found in surfactant isolated from normal animals. SP-B is also found in club cells (Clara), but the function of SP-B associated with club cells is not known. In SP-B deficiency, there is also aberrant processing of SP-C, which results in the secretion of a partially processed 12 kDa form of SP-C.
In summary, SP-B is a hydrophobic protein critical for the function of surfactant, and a total deficiency in SP-B is a cause of respiratory distress in the newborn. This form of respiratory distress is not responsive to surfactant replacement therapy and requires lung transplantation.
Surfactant Protein C
SP-C is an extremely hydrophobic protein that is unique to surfactant and alveolar TII cells. SP-C is expressed only in the lung and is a highly specific marker for identifying TII cells. However, the precise physiologic function of this protein is not completely understood. The fully processed form of SP-C is a 35–amino acid lipopeptide that has two palmitates attached as thioesters at amino acids C5 and C6. The segment between residues 13 and 28 forms a hydrophobic α-helix containing aliphatic amino acids, mainly valine. This α-helix is the membrane-spanning portion of SP-C and is extremely stable. In most species, there are two positively charged amino acids, lysine at position 11 and arginine at position 12, which appear to be necessary to move the protein from the ER into the Golgi network, where it is palmitoylated. The precise orientation of the palmitates relative to the valine-rich α-helix is not fully resolved, but both regions are probably important for interactions with phospholipid bilayers or monolayers. SP-C can mix with phospholipids and promote spreading and fusion of phospholipids. SP-C inserts into the monolayer and is squeezed out of the surface monolayer only at relatively high film pressures (>55 mN/m). Presumably, SP-C is important in organizing the phospholipids during the respiratory cycle. SP-C is thought to stabilize the surface film and minimize film collapse. Unlike SP-B, SP-C does not appear to interact with SP-A and is not critical for the formation of tubular myelin. SP-C is found in all preparations of natural surfactant, and a recombinant form of SP-C is used in one type of surfactant replacement therapy.
In humans, there is one gene for SP-C that is located on human chromosome 8. Like SP-B, SP-C is synthesized as a larger precursor form of 21 kDa and processed by proteolytic cleavage of both N-terminal and C-terminal fragments. Most of the posttranslational processing has been completed before SP-C arrives at the lamellar body. There is apparently some interaction between the processing of SP-B and SP-C because, in hereditary deficiency of SP-B, an aberrantly processed 12 kDa form of a precursor SP-C accumulates in TII cells and in alveolar fluid. The SP-C promoter has been an important tool for overexpressing a variety of transgenes in TII cells in mice.
The physiologic role of SP-C is still not completely known. In one strain, SP-C null mice appear normal until about 6 months of age, at which time they develop chronic pneumonitis and air space enlargement. This demonstrates that SP-C is not absolutely necessary for surfactant function. However, in another strain, mice develop chronic pneumonitis sooner, within 3 months. The genetic background and disease-modifying genes are, therefore, likely involved in clinical manifestations of SP-C deficiency and mutations. Some SPC mutations in humans also produce a misfolded protein that accumulates and causes ER stress in TII cells and a chronic interstitial lung disease. These observations provide the background for investigating genetic variants in SP-C as a cause of idiopathic interstitial lung diseases, which is discussed later.
In summary, SP-C is a hydrophobic protein that inserts into the surfactant monolayer but does not appear to be critical for surfactant function. However, mutations in this protein and total absence can result in interstitial lung disease. This is the one surfactant protein that is restricted to TII cells.
Surfactant Protein D
SP-D is a calcium-dependent lectin and an important component of innate immunity. Recombinant SP-D has even been proposed as a therapeutic agent in the treatment of pulmonary infections. Serum SP-D is increased in interstitial lung diseases and may predict outcome in lung transplant recipients. In humans, the gene for SP-D is located near the locus of SP-A on chromosome 10. SP-D is a collagenous glycoprotein with a complex but highly ordered tertiary structure (see Fig. 8-6 ). The primary structure consists of four domains. The N-terminal segment contains cysteines that form interchain disulfide bonds that cross-link subunits to form covalent oligomeric structures. The adjacent collagen-like region promotes the formation of noncovalent trimers, imparts a rigid longitudinal structure to the molecules, and organizes the spatial distribution of the C-terminal CRDs. The coiled-coil motif of the neck contributes to the spatial organization of the CRD domains. The C-terminal CRD domain contains the calcium and carbohydrate binding sites. The crystal structure of the CRD and neck region of SP-D has shown a distinct spatial distribution of the three CRDs, has defined the carbohydrate binding pocket, and has allowed for computer docking studies to demonstrate the importance of specific vinyl hydroxyl groups in sugars for binding. Identical monomers of SP-D assemble as trimers and then combine to form the final dodecamer, a large symmetric cruciform-shaped molecule with a distance of about 100 nm between the terminal CRDs, about five times larger than SP-A. The CRD unit is primarily responsible for the multivalent binding to surface ligands on microorganisms. As in the case of SP-A, the full oligomerization of SP-D appears to play an important role in maintaining biologic potency that may result from specific spatial cross-linking of ligands, as well as amplification of relatively weak interactions through multiple binding sites.
Conceptually, SP-D should be thought of as a protein distinct from the surfactant system. SP-D binds the phospholipids of surface-active material weakly and is mostly soluble in alveolar fluid. However, SP-D does bind two lipids with carbohydrate motifs, phosphatidylinositol and glucosylceramide, but not phosphatidylcholine. The location of SP-D in the lung also suggests that it is not directly involved with the surfactant system. SP-D is found in ER of TII cells and in the secretory granules of club cells or nonciliated bronchiolar cells but not in lamellar bodies of TII cells or in tubular myelin. SP-D is highly expressed in the hyperplastic TII cells present in interstitial lung diseases. Like SP-A, SP-D is highly expressed in conducting airways of rodents but sparsely in the major conducting airways in humans. SP-D is also present on many other mucosal surfaces.
SP-D is an important host defense molecule and binds a variety of organisms, usually through its CRD domain. In terms of monosaccharide binding, SP-D has a preference for maltose, glucose, and mannose. The binding of SP-D to organisms can be altered by physiologic glucose concentrations, and impaired binding in the presence of glucose may be important in diabetics. SP-D binds influenza A, inhibits hemagglutination, and inhibits infection. It is interesting to note that the annual severity of influenza infections is related to the ability of SP-D to bind to the circulating strains of influenza. Strains with less SP-D binding such as the 1918 H1N1 strain and the pandemic 2009 strain are clinically more virulent. Although studies on the interaction of SP-D with viruses have been reported for only a few viruses, it is likely that SP-D binds to many respiratory viruses. SP-D also binds to bacteria and should be considered an important molecule in host defense. SP-D binds both major components of gram-positive cell walls, peptidoglycan, and lipoteichoic acid. SP-D also binds to LPS and gram-negative bacteria with the rough form of LPS and aggregates these bacteria. SP-D also is likely important in defense against fungal infections. SP-D binds to Aspergillus fumigatus conidia and histoplasma and enhances their phagocytosis and killing. SP-D also binds to and agglutinates Saccharomyces cerevisiae, and the cell wall ligand for binding is β (1→6) glucan. This binding is inhibited by pustulan, an extremely effective competitive inhibitor of SP-D. SP-D also binds Alternaria, a common mold and aeroallergen, and SP-D may be important in clearing a variety of inhaled fungal spores. Finally, SP-D agglutinates Mycobacterium tuberculosis but inhibits its phagocytosis by human macrophages. Once in macrophages, SP-D promotes phagosome-lysosome fusion and thereby intracellular killing of mycobacteria.
SP-D has been reported to bind to several different receptors on macrophages and to stimulate microbicidal metabolism, as indicated by the production of reactive oxygen species. Rat SP-D enhances oxygen radical production by alveolar macrophages but not peritoneal macrophages. However, there remain concerns that, in the early studies, the stimulation of macrophages by SP-D could be due to endotoxin contamination. SP-D is also involved in the clearance of apoptotic cells. SP-D binds to apoptotic cells and facilitates their ingestion by macrophages through the calreticulin and CD91 complex.
The phenotype of the SP-D knockout mouse was unexpected from prior in vitro studies. The knockout mouse shows an increase in the extracellular pools of surfactant and an accumulation of large foamy macrophages with excess metalloprotease activity, which results in alveolar wall destruction and subsequent air space enlargement. The implications are that SP-D assists in clearance of surfactant and regulates macrophage function. In addition, a large number of apoptotic cells are found in the air spaces and lavage fluid of SP-D null mice, indicating that SP-D has an important role in the clearance of apoptotic cells. SP-D-deficient mice are also susceptible to infection with various microbes, including influenza A virus and Aspergillus.
The interactions of SP-D and SP-A with organisms and phagocytic cells are complex, and the clear identification of the receptors for these proteins is awaited in order to sort out apparently conflicting observations. The interaction will be affected by the organism, growth cycle of the organism, phagocytic cell, and state of activation of the phagocytic cell, as well as the source of SP-A or SP-D and the presence or absence of contaminating endotoxin or TGF-β in the surfactant protein preparation.
In summary, SP-D is a host defense protein like mannose binding lectin and should not be considered as part of the surfactant system. The major functions of SP-D are the clearance of heavily glycosylated viruses such as influenza and the removal of apoptotic cells.
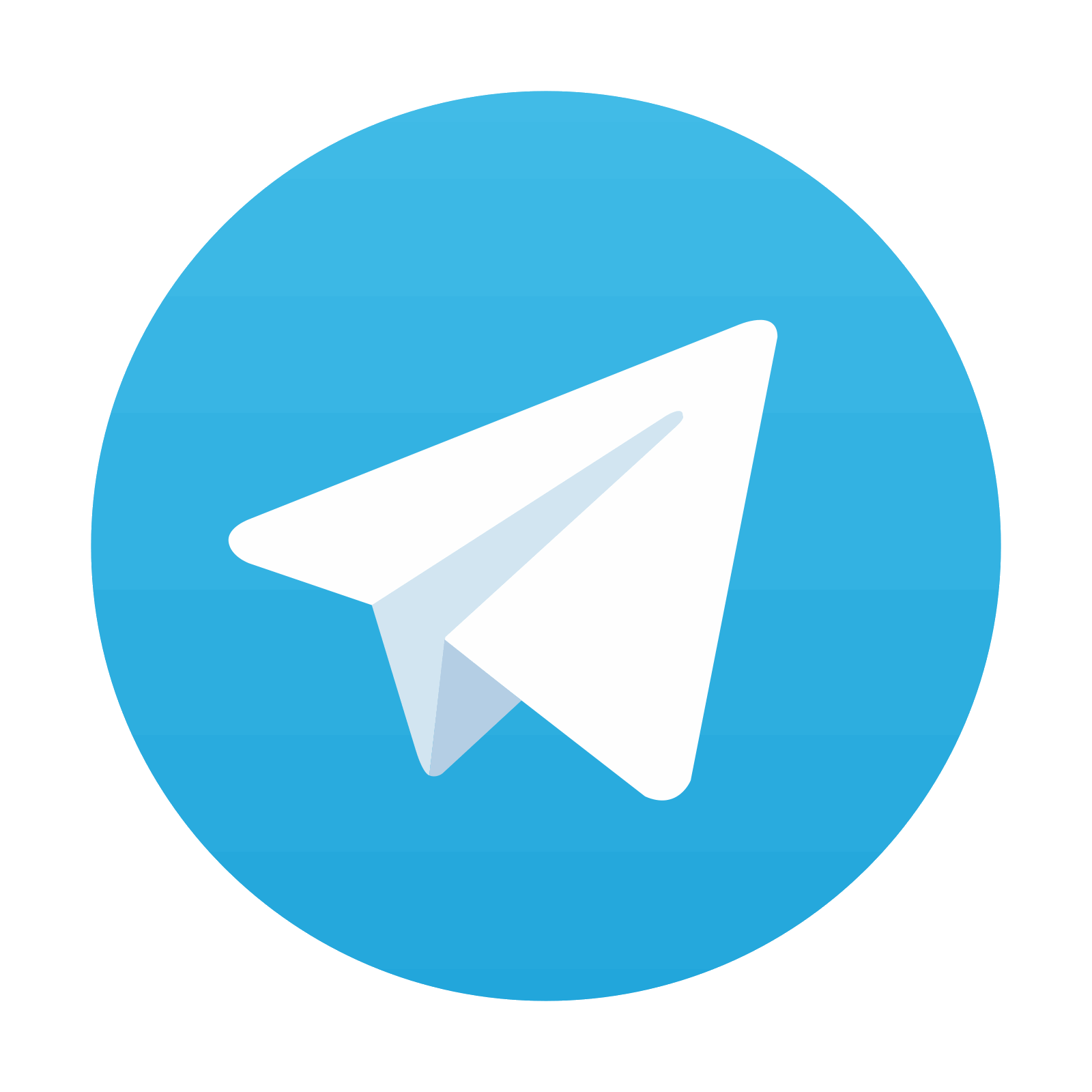
Stay updated, free articles. Join our Telegram channel
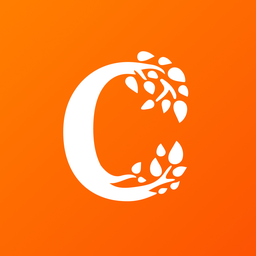
Full access? Get Clinical Tree
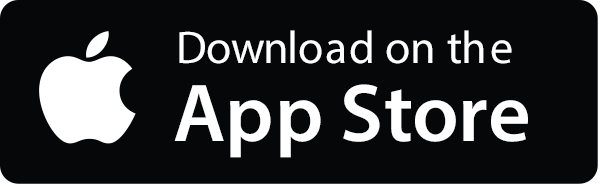
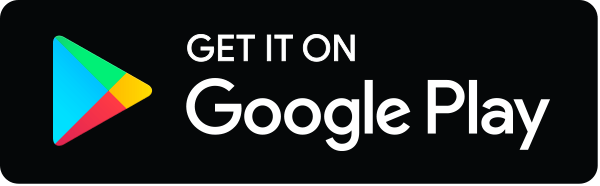