Aging of the Respiratory System
The fastest growing segment of the US population consists of individuals of age 65 and older. In the 2010 census, this segment comprised 16% of the population. Since the 1950s, the median age of the US population has increased by 20 years.
The largest decrements in age-adjusted death rates have been occurring in older patients. With the increasing longevity of human population, it is necessary to understand the effects of aging on the respiratory system in healthy people. Perhaps more importantly, because the age-related decrements in respiratory system function can be unmasked by disease, it is critically important to point out that the aged: (1) have an enhanced predisposition to lung disease; (2) have a decreasing reserve of respiratory system function that decreases their ability to cope with the stresses of illness, injury, and surgery; and (3) may have differing responses to therapy when compared with their younger counterparts.
Even in individuals who enjoy apparently good health, there are measurable decrements in function of the respiratory system with age. These changes occur progressively as a healthy individual grows older and are most marked beyond 60 years of age. Cross-sectional studies show clear differences between elderly and young persons with regard to the structure and function of the components of the respiratory system (Table 19-1). As we will see, however, caution must be exercised in ascribing observed changes to age alone. It is also necessary to be aware that longitudinal studies of “healthy” individuals followed to old age are essentially not available. Where appropriate, methodologic problems in the available cross-sectional studies are described.
TABLE 19-1 Respiratory System: Functional Divisions and Changes with Aging
Although age-associated changes can be measured easily by objective testing, it is important to note that the routine activities of healthy elderly persons are not limited by the decreasing respiratory system function. However, whereas youthful persons have a marked excess of functional capacity over the amount required to meet metabolic needs at rest or with stress (physiologic reserve), the respiratory system draws on this reserve as its function declines with age. Thus, the physiologic reserve, especially for alveolar gas exchange, is reduced with aging. This leaves elderly individuals vulnerable to stresses, diseases, and injuries that are weathered much more easily in the young.
ARE CHANGES IN THE RESPIRATORY SYSTEM CAUSED BY AGING OR RELATED TO AGE IN OTHER WAYS?
For decades, the phenomena explained purely by aging have been required to satisfy specific principles. Roughly according to Hayflick,1 the changes must be (1) intrinsic (as opposed to environmentally mediated); (2) universal; (3) progressive; and (4) usually detrimental to the organism. Developing an understanding of changes to the lung over time is complicated by the fact that the lung is an open system that is exposed to environment. As such, it is assaulted by respiratory infections and by a constant barrage of particulates and other air pollutants. In addition, the lungs are commonly exposed to tobacco smoke, to occupational dusts and fumes, and to effects of aspiration. At times, environmental influences are inextricably involved in the changes that are observed in the respiratory system over time. However, it is important to draw a distinction between alterations purely related to aging and those associated with older individuals. Purely age-related changes are biologic phenomena and irreversible. Other alterations associated with increasing age have the potential to be preventable, treatable, and/or reversible.
CHANGES IN THE UPPER AIRWAY
There is a clearly increased risk of aspiration in the elderly that is thought to be a consequence of a number of age-related factors, including comorbid illnesses and debility, medications, and the aging process itself. With regard to purely age-related changes, attention has been focused upon the cough and swallowing reflexes, both of which are protective against aspiration. In the past, these reflexes have been thought to be controlled primarily by the brainstem, but there is now evidence that cortical and subcortical structures play critical roles in their control.2 By their nature, reflexive cough and swallowing activate both sensory and motor areas in the cortex. The sensory component, including the sensory cortex in reflexive circuits, seems to be more vulnerable to aging than the motor component, including the motor cortex. Therefore, strategies to restore effective cough and swallowing reflexes should be focused upon compensations of sensory components.
Variable amounts of aspiration of both oropharyngeal contents (food particles, saliva, and oropharyngeal organisms), and also gastric contents (food particles and gastric secretions including gastric acid), are increasingly common with aging. Aspiration is particularly common in the very old. Because of the sensory alterations discussed earlier, aspiration in elderly individuals can be associated with few, or no, symptoms.3
A potentially important contributor to aspiration is gastroesophageal reflux. In accordance with the earlier discussion of sensory impairments, reflux esophagitis severity increases with age while heartburn severity decreases with age.4 Consequences of gastroesophageal reflux may be exacerbated by esophageal motor abnormalities associated with aging. Recurrent aspiration of gastric contents in the elderly can lead to airway inflammation, bronchiectasis, and pneumonia.
STRUCTURAL CHANGES IN THE LUNG
Studies of the aging lung have shown changes in shape, with increase in anteroposterior diameter that lead to a “rounding” shape of the lung. These changes are presumably secondary to changes in the shape of the surrounding thoracic cage that are very common after the age of 75. In a study of 100 chest radiographs from individuals ranging in age from 75 to 93 years, 25% had severe kyphosis (>50 degrees) and 43% had moderate kyphosis (35–50 degrees) from vertebral fractures.5
CONDUCTING AIRWAYS
The conducting airways consist of the air passages from mouth to the level of respiratory bronchioles. Their volume comprises the anatomic dead space, and their geometry is a primary determinant of airway resistance. The larger cartilaginous airways show a modest increase in size with age, resulting in slight but probably functionally insignificant increase in anatomic dead space. Although calcification of cartilage in the walls of the central airways and hypertrophy of bronchial mucus glands is seen in advanced age, these changes in the extraparenchymal conducting airways appear to have little or no physiologic significance.
Ciliary motility is significantly decreased in subjects over the age of 60, which likely further increases the risk of lower respiratory infection and inflammation.6
LUNG PARENCHYMA
After the age of 30 or 40, the respiratory bronchioles and alveolar ducts undergo progressive enlargement (Fig. 19-1). This change has been termed “ductectasia” because of the prominent finding of enlargement of alveolar ducts.7 The proportion of the lung made up of alveolar ducts increases, and alveolar septa become shortened, leading to a “flattened” appearance of the alveoli. With the change in geometry, the distance between alveolar walls (known to morphologists as the mean linear intercept, or MLI) increases, while the surface-to-volume ratio of the lung decreases.8 The age-related enlargement of the terminal respiratory units also produces a decrease in the percentage of parenchymal air contained within the alveoli.9 The net result of these structural changes is that the alveolar surface area decreases by approximately 15% by age 70.
Figure 19-1 Histologic changes in the aging lung. A. Normal lung of a 36-year-old woman. B. Lung of a 93-year-old woman. In (B), the alveolar ducts are dilated, and shortening of inter-alveolar septa is observed. (Used with permission of the Mayo Foundation; photomicrographs used with permission of Charles Kuhn III, MD.)
Pulmonary emphysema is also characterized by an increase in the size of terminal airspaces, an increase in MLI, and a decrease in surface area; however, destruction of alveolar septa with fusion of terminal airspaces is a defining characteristic of emphysema. There have been some reports of emphysematous lesions in aged lungs, but it is not certain that smokers were excluded from these studies. Since the fate of individual alveolar septa during the aging process has been somewhat controversial, some have referred to the histologic changes in aged lungs as “senile emphysema.” A National Heart, Lung, and Blood Institute Workshop on the definition of emphysema10 weighed the available evidence, and decided not to include age-related changes in the lung parenchyma under the definition of emphysema. To simplify terms and avoid confusion, they recommended use of the term aging lung to apply to the uniform airspace enlargement that develops with increasing age. Despite the continued use of “senile emphysema,” this term should be avoided.
Computed tomography of the chest in individuals over 75 years of age has shown a surprising prevalence (60%) of a subpleural basilar reticular pattern that was absent from images from a control population that was less than 55 years old. Lung cysts were seen in 25% of the elderly subjects, but none of the controls.11 These findings are usually associated with interstitial lung disease, but in the elderly they seem not to reflect a clinically relevant disease process. Caution must be exercised to avoid overreacting to similar image findings in older patients.
CHANGES IN MECHANICAL PROPERTIES OF THE LUNGS
Both the Lungs and chest wall are elastic. The resting volume of excised lungs is smaller than that contained within an intact thoracic cage, because the lungs are held at an increased volume by the outward recoil forces of the chest wall. Thus, in the intact thoracic cage, the lungs exert an inward recoil force. The retractile force of the lungs, or the “elastic recoil,” can be measured during life by estimating the pleural pressure with an esophageal balloon at progressively decreasing lung volumes from total lung capacity to functional residual capacity (FRC), when the airways are open and there is no air flow. The negative pleural pressure is generated by the lungs’ elastic recoil forces.
The pressure measurements may be displayed on a pressure–volume diagram (Fig. 19-2). Figure 19-2 compares, at the same volume, the elastic recoil pressures of a young man, a normal elderly adult, and a patient with emphysema. The normal elderly individual and the patient with emphysema, both have a greater decrease in elastic recoil pressure than does a young person.12 This is reflected in the leftward shift of their pressure–volume curves.13–16 This loss of elastic recoil is the physiologic hallmark of emphysema. However, emphysema is characterized by a much greater loss of elastic recoil than is caused by aging alone.
Figure 19-2 Static pressure–volume curves of the lungs. Static recoil pressure, expressed as transpulmonary pressure measured at various lung volumes, is plotted against lung volume on the ordinate. Note that at any lung volume, the recoil pressure is less in the aged individual than in the young, normal control, resulting in a pressure–volume curve that is shifted upward and to the left. For comparison, a curve for a patient with emphysema is shown. In emphysema, recoil pressures are reduced much more, and lung compliance (the slope of the pressure–volume relationship) is clearly abnormal. (Reproduced with permission from Pride NB. Pulmonary distensibility in age and disease. Bull Physiopathol Respir. 1974;10(1):103–108.)
There has been some disagreement regarding the effects of aging on lung compliance (Δ volume/Δ pressure; that is, the slope of the pressure–volume relationship Fig. 19-2). The question is whether there is a parallel leftward shift of the pressure–volume curve with aging (no change in compliance), or, instead, a steeper slope in addition to a shift (indicating an increase in compliance), as seen in emphysema. In aged individuals, the static pressure–volume curve is slightly steeper and is more concave in relation to the pressure axis. However, there is a general agreement that changes in lung compliance with aging are not physiologically significant.
Two forces in the lung parenchyma are responsible for producing the elastic recoil of the lungs. The greatest part of the elastic recoil forces is provided by the surface tension at the curved air–fluid interface of the small airways and alveoli. The second retractive force is that produced when the fibrous skeleton of the lung (primarily the elastic fibers) is stretched.
CHANGES IN SURFACE FORCES
Most of the loss in lung recoil with age is likely to be related to the decrease in lung surface area with age The loss of surface area that accompanies aging can be expected to reduce the area of gas–liquid interface, resulting in a decrease in the surface tension forces and, ultimately, a decrease in the total elastic recoil of the lung. Whether it is due to loss of air–liquid interface or to changes in lung structural macromolecules the reduced elastic recoil has important consequences for the function of the intraparenchymal airways and, ultimately, on alveolar gas exchange and forced expiratory flow (see “Pulmonary Function Tests”).
CHANGES IN STRUCTURAL MACROMOLECULES
Weibel has produced elegant studies of the “integral fiber continuum” that extends from the hila to the pleura. This consists of axial fibers extending to the alveolar septae, and septal fibers extending to the pleura.17 Although most proteins in the lung turn over relatively rapidly, the structural proteins, elastin and collagen, in the lung fibrous network provide a very stable, long-lived skeletal structure for the lung.
Elastic fibers consist in large part of an extremely hydrophobic, highly cross-linked, and very elastic macromolecule (elastin). These fibers are thought to contribute substantially to lung elasticity. Analysis of whole lungs has revealed that the elastin content actually increases (rather than decreases) with age. More recent evidence indicates that the increase in lung elastin with age is accounted for by an increase in pleural elastin; parenchymal elastin does not change.
Careful studies of the elastic fibers in the lung parenchyma have shown that they are remarkably stable following postnatal lung growth. Certain biochemical changes in very long-lived proteins (change of amino acids into their mirror-image structures, or racemization) provide a type of “biological clock” that permits an estimate of the time that has elapsed since the proteins were synthesized. Because of the constraints of the protein synthetic mechanisms, only L-amino acids are incorporated into newly synthesized proteins. With the passage of years at body temperature, however, there is a readily measurable accumulation of D-aspartic acid. When all of the lung proteins are examined together, minimal D-aspartic acid is found. In purified lung elastin, however, there is an age-related accumulation of D-aspartic acid,18 indicating that lung elastin is turning over very slowly if at all (Fig. 19-3). It has also been possible to estimate lung elastin turnover by measurement of the incorporation into elastic fibers of carbon 14 (14C) from atmospheric nuclear weapons testing. For example, individuals who completed their postnatal lung growth prior to the nuclear age show no excess 14C in their lung elastin, indicating absence of new elastin synthesis. In contrast, an appropriate excess of 14C is found in the lung elastin of individuals whose lungs were growing in the post–weapons testing era. Modeling of the radiocarbon data indicates that the “mean carbon residence time” in elastin is 74 years.18
Figure 19-3 Longevity of human lung parenchymal elastin, as evidenced by in vivo racemization of aspartic acid. Each pair of bars shows results from two individuals with greatly differing ages at time of death. Step 0 of elastin purification represents whole lung parenchyma, while step 5 is purified elastin. D-aspartic acid detected in the 6-year-old specimen can be attributed to racemization that occurs during the analytical procedures, whereas, the difference in prevalence of D-aspartic acid between the young and old individual has resulted from racemization in vivo. Note that results from whole-lung hydrolysates (step 0) are similar for both specimens, reflecting their composition of proteins, having predominantly rapid turnover. However, purified elastin from the oldest specimen has racemized extensively in vivo, indicating that it was synthesized many decades before death. These data for elastin agree well with results for other very long–lived proteins. (Reproduced with permission from Shapiro SD, Endicott SK, Province MA, et al. Marked longevity of human lung parenchymal elastic fibers deduced from prevalence of D-aspartate and nuclear weapons–related radiocarbon. J Clin Invest. 1991;87(5):1828–1834.)
Taken together, the amino acid racemization and radiocarbon data indicate that lung parenchymal elastin is stable over the human life span, and it appears that the elastin content of the lung parenchyma not only does not change with age, but the individual fibers persist for at least many decades.
Other studies of lung elastic fibers have shown changes in the location and orientation of individual fibers with age as well as changes in the cross-linking of elastin. Thus, some authors have suggested that remodeling of the lung architecture may occur without replacement of elastic fibers. In any case, at the present time, the age-related changes in connective tissue do not provide a sufficient explanation for the decrease in elastic recoil forces observed in the elderly.
Studies of collagen in alveolar walls of humans, as measured by hydroxyproline, have failed to show a consistent change in its quantity during aging.19 Although human studies have not been done, studies in rodents and birds suggest that lung collagen fibers, like elastic fibers, are very long-lived.20 Finally, although some qualitative changes in collagen during aging have been described (decreases in solubility and increases in intermolecular cross-links), these appear to have no relationship to changes in lung elastic recoil.
Although convincing evidence for very slow compensatory human lung growth (over many years) has been provided following pneumonectomy,21 under more ordinary circumstances it appears that the lung adapts to changing dimensions of thorax by realignment of its fibrous skeleton rather than by molecular remodeling.
CHANGES IN CHEST WALL
There is good evidence that the chest wall becomes more rigid with advancing age.22 As may be seen in Figure 19-4, the static pressure–volume curve of the chest wall is shifted to the right and is less steep with increasing age. The articulations of the ribs with the sternum and the spinal column may become calcified, and the compliance of the rib articulations decreases. The changes in rib articulations may be compounded by the development of kyphosis due to osteoporosis. The decreasing compliance of the chest wall demands greater work from the respiratory muscles. For example, in a 70-year-old person, approximately 70% of the total elastic work of breathing is expended on the chest wall, whereas this value is 40% in a 20-year-old person.
Figure 19-4 Static compliance relationships of the components of the respiratory system. (L, lungs; W, chest wall; RS, total respiratory system.) A. A 20-year-old man. B. A 60-year-old man. Note that the static compliance of the chest wall is substantially decreased (reduced slope) in the older individual, while functional residual capacity (the resting volume of the respiratory system, or the point at which the pressure gradient across the respiratory system is zero) increases. As in Figure 18-2, it is also apparent that the static recoil pressure of the lungs is reduced in the older subject. (Data from Mittman C, Edelman NH, Norris AH, et al. Relationship between chest wall and pulmonary compliance and age. J Appl Physiol. 1965;20:1211–1216; and Turner JM, Mead J, Wohl ME. Elasticity of human lungs in relation to age. J Appl Physiol. 1968;25:664–671.)
Figure 19-4 also demonstrates that the compliance of the total respiratory system decreases with age because the decrease in lung elastic recoil is outweighed by the changes in the mechanical properties of the chest wall.
CHANGES IN MUSCLES OF RESPIRATION
Age-related changes in nonrespiratory skeletal muscle include decreased work capacity due to alterations in the efficiency of muscle energy, metabolism, atrophy of motor units, and electromyographic abnormalities. Based upon lessons learned with other skeletal muscles, it appeared likely that age-related abnormalities in respiratory muscles also would be found.
An early study by Black and Hyatt appeared to confirm age-related decrements in respiratory muscle function by measuring maximal inspiratory pressure (PImax) and maximal expiratory pressure (PEmax) in 120 normal individuals (both smokers and nonsmokers) between the ages of 20 and 70.23 Maximal respiratory pressures in females were 65% to 70% of those in males. No significant age-related changes were observed in individuals under the age of 55. Trends toward reduced maximal respiratory pressures with age were seen in both genders and with both PImax and PEmax. With the number of males studied, the change with age in PImax was not statistically significant for male gender.
More recently, McElvaney and coworkers came to a different conclusion in a similar study of 104 healthy individuals over the age of 55.24 They found a large variation in maximal respiratory pressures from individual to individual (as by Black and Hyatt), but no significant correlation with age. In contrast, in a third population of 160 healthy individuals who ranged in age from 16 to 75 years, Chen and Kuo found significant gender differences in maximal respiratory pressures as well as trends toward decrements with age for PImax and PEmax in both genders. The age-related change in PEmax in males was not statistically significant with the sample size studied. When the 40 individuals of both genders in the youngest age group (16–30 years) were compared with the 40 individuals in the oldest group (61–75 years), the decrement in PImax was 32% to 36%, while the decrement in PEmax was 13% to 23%.25 Representative findings for maximal respiratory pressures in women are illustrated in Figure 19-5.
Figure 19-5 Representative variations in maximal respiratory pressures with age among women. Inspiratory and expiratory measurements were made at residual volume and total lung capacity, respectively. Maximal inspiratory pressure (open bars) and maximal expiratory pressure (hatched bars). Error bars are standard errors of the mean. Although quantitatively moderate, variations with age were statistically significant for both measurements. (Data from Chen H-S, Kuo C-S. Relationship between respiratory muscle function and age, sex, and other factors. J Appl Physiol. 1989;66(2):943–948.)
Chen and Kuo also measured inspiratory muscle endurance against a resistive load, and found significant decrements with age. Physically active men had greater inspiratory muscle endurance than sedentary men.
In summary, it appears that when populations of healthy individuals of widely differing ages are studied, moderate age-related decrements in respiratory muscle strength and endurance can be found. These studies usually define healthy only by the absence of disease and do not control for physical activity. They are complicated by marked interindividual variability, and longitudinal studies have not been reported. Continuous respiratory muscle activity may have a training effect that leads to better preservation of respiratory muscle function when compared with other skeletal muscles. Finally, physical activity may have an additional training effect that enhances inspiratory muscle endurance in all age groups.
CONTROL OF BREATHING
In young individuals, minute ventilation is matched with metabolic demands. As a result, arterial blood gas values remain stable throughout a wide range of activities from rest to strenuous exertion, while oxygen consumption and carbon dioxide production are varying widely. Similarly, when the efficiency of gas exchange is diminished by lung disease or congestive heart failure, appropriate increases in minute ventilation minimize the resulting hypercapnia and/or hypoxemia in healthy young individuals. The ventilatory control system is described in detail in Chapter 11.
Ventilatory control mechanisms are typically tested by stressing the respiratory system, by inducing either hypoxemia or hypercapnia while monitoring ventilatory parameters (and often cardiac parameters as well). Such tests have shown striking differences between young and elderly individuals in both ventilatory and cardiac responses.
DIMINISHED VENTILATORY RESPONSE TO HYPERCAPNIA
Kronenberg and Drage compared the ventilatory responses to hypercapnia in eight young (mean age, 25.6 years) and eight elderly (mean age, 69.6 years) individuals.26 During the tests, the subjects were asked to rebreathe 5% CO2, while their PAO2 was held above 200 mm Hg by supplemental oxygen to eliminate hypoxic ventilatory drive. Measurements were made while PAC2 was allowed to rise to 65 mm Hg. Although there was considerable individual variation and some overlap between the groups, the elderly individuals had a significantly diminished ventilatory response to hypercapnia, measured as the slope of the relationship between ventilation and PAC2.
DIMINISHED VENTILATORY RESPONSE TO HYPOXIA
When Kronenberg and Drage measured the ventilatory response to hypoxia at constant CO2, they found even more striking differences between the young and elderly subjects (Fig. 19-6). For example, the ventilatory response to a PAO2 of 40 mm Hg was uniformly smaller in the older subjects, and there was no overlapping between the groups.26 The mean minute ventilation at a PAO2 of 40 mm Hg was 40.1 and 10.2 L/min in the young and old groups, respectively.
Figure 19-6 Variations with age in ventilatory responses to hypoxia. Eight normal men aged 64 to 73 (circles) and eight controls aged 22 to 30 (squares) were subjected to isocapnic progressive hypoxia by a rebreathing method. Data values are means, with standard errors of the mean shown by the error bars. Note that the ventilatory responses differ strikingly between the elderly individuals and the controls. (Reproduced with permission from Kronenberg RS, Drage CW. Attenuation of the ventilatory and heart rate responses to hypoxia and hypercapnia with aging in normal men. J Clin Invest. 1973;52(8):1812–1819.)
DIMINISHED OCCLUSION PRESSURE RESPONSES
Peterson et al. confirmed the previously discussed observations and have shown that the differences in responses of elderly subjects to both hypercapnia and hypoxia are due to a lesser increase in tidal volume while the ventilatory rate increases normally. Since this observation could be caused by differences in respiratory muscle strength or increases in chest wall stiffness, the authors also measured airway occlusion pressures, which are valuable indices of respiratory drive that are not affected by respiratory muscle strength or respiratory mechanics.27 The measurement (P100) is the negative pressure at the mouth, measured 100 ms after the start of inspiration against an occluded airway. The occlusion pressure responses to both hypoxia and hypercapnia (Fig. 19-7) were significantly reduced in the 10 elderly subjects studied by Peterson (mean age, 73.3 years) when compared to nine young control subjects (mean age, 24.4 years). Although the elderly individuals had reduced respiratory muscle strength (mean, 24% lower maximal static inspiratory pressure), the differences in occlusion pressure persisted when normalized for these differences.
Figure 19-7 Variations with age in occlusion pressure responses to hypoxia and hypercapnia. Data shown are slopes of relationships between occlusion pressure responses and either SaO2 or end-tidal PCO2; error bars are standard errors of the mean. Elderly individuals had significantly diminished occlusion pressures in response to both hypoxia and hypercapnia. Both differences were significant, with p < 0.01. (Reproduced with permission from Peterson DD, Pack AI, Silage DA, et al. Effects of aging on the ventilatory and occlusion pressure responses to hypoxia and hypercapnia. Am Rev Respir Dis. 1981;124(4):387–391.)
The previously mentioned seminal studies of ventilatory control have been discussed in some detail out of respect for their ground-breaking conceptual importance. Subsequent studies have created some confusion that appears likely to be due to methodologic differences, ages of subjects, and small sample sizes. However, in 14 of 16 studies reviewed by Lalley, ventilatory responses in the elderly were found to be abnormal.28
In summary, the reduced responsiveness in tidal volume to either hypoxemia or hypercapnia with age is apparently due to a reduced responsiveness of ventilatory drive or neural output from the respiratory center. It has not been determined whether the diminished ventilatory drive results from altered chemoreceptor function or from altered function of the respiratory center. Kronenberg and Drage favored altered receptor function based on their observation that elderly subjects responded to an alveolar oxygen tension of 40 torr with only an 11% increase in heart rate, whereas the young subjects responded with a 34% increase.
RESPIRATORY LOAD COMPENSATION AND DYSPNEA
Reflex compensation for a change in respiratory mechanical load (as in lung disease, changes in posture, and mouth versus nose breathing) normally serves to maintain ventilation constant during the change. Akiyama et al. measured responses to inspiratory flow-resistive loading in young and elderly individuals and found significant differences.29 In the young control group, inspiratory loading resulted in an increase in P100 at each level of induced hypercapnia, such that inspiratory loading did not change the ventilatory response to hypercapnia when compared with unloaded responses. In marked contrast, the P100 in the elderly group did not change when an inspiratory load was applied. In the absence of a compensatory change in ventilatory drive, ventilatory responses to hypercapnia were reduced during inspiratory loading in the elderly group.
At each level of PCO2, the intensity of perceived dyspnea in response to inspiratory loading was greater in the elderly than in the control group. Thus, the sensation of dyspnea was intact or enhanced in the elderly subjects, while their compensatory responses were reduced. This suggests the possibility that, elderly individuals may complain of a greater dyspnea than younger individuals with similar pathophysiologic deterioration.
SENSITIVITY TO RESPIRATORY DEPRESSION BY OPIOIDS AND SEDATIVES
Older individuals are substantially more sensitive to respiratory depression by opioids and sedatives. This phenomenon demands extra vigilance when these medications are prescribed in nonintubated elderly patients. The enhanced drug effect has been shown to be multifactorial, and the various mechanisms involved are beyond the scope of this chapter.30–32 However, the dangers associated with higher levels and more prolonged effects of these drugs are real and potentially catastrophic.
PULMONARY CIRCULATION
Invasive physiologic studies of pulmonary artery catheterization have typically been biased by including only subsets of patients whose signs and symptoms led to referral for heart catheterization and who, therefore, may not be representative of a “healthy” cohort. Further, age-related changes in the pulmonary circulation are difficult or impossible to separate from changes due to heart disease or age-related changes in cardiac function.
Ehrsam et al. reported a retrospective analysis of right heart catheterization studies performed in 125 asymptomatic subjects who ranged from 14 to 68 years of age.33 Small increases in right atrial, pulmonary artery, and pulmonary artery wedge pressures observed in the highest age group disappeared when values were adjusted for sex, weight, and height. No significant age-related changes were found in cardiac output, stroke volume, or oxygen uptake. Age explained 10% or less of the total variation in the hemodynamic and pressure variables when assessed by multiple regressions. During supine exercise with a bicycle ergometer, however, pulmonary artery and wedge pressures increased with age, particularly in subjects over the age of 45. The changes were highly significant with age accounting for 12% to 30% of the total variation when assessed by multiple regressions. Finally, pulmonary artery resistance showed a highly significant increase with age, whether measured at rest or during exercise, with age contributing 12% to 27% to the total variation in pulmonary artery resistance. Although the cohort studied were all asymptomatic and ambulatory, it is possible that silent coronary artery disease was present in some of the subjects, and the prevalence of coronary artery disease can be expected to increase with age. Moreover, younger patients tended to be referred for evaluation of a heart murmur, whereas the older patients were referred for “pulmonary investigation” that included coin lesions, hilar lymphadenopathy, “previous pulmonary infiltrates,” and smoke inhalation. Cigarette smoking history was not discussed. Thus, it is not certain that the younger and older patients were strictly comparable.
More recently, Davidson and Fee reported the results of right-heart catheterization at rest in 47 normal subjects who were free of coronary disease and had normal left ventricular systolic function.34 Smokers were included. The investigators found highly significant but quantitatively modest age-related increases in mean pulmonary artery pressure, pulmonary vascular resistance, and pulmonary/systemic vascular resistance ratio, but they found no age-related differences in pulmonary artery wedge pressure. The authors felt that the most likely explanation for the age-related changes in pulmonary artery pressure and pulmonary vascular resistance was a primary abnormality of the pulmonary vascular bed, but they could not exclude the effects of subtle abnormalities in left ventricular function.
In summary, studies of pulmonary hemodynamics with aging are limited by retrospective design, bias in patient selection, and potential effects of smoking. Minor increases in pulmonary vascular resistance and age-related increases in pulmonary artery wedge pressure during exercise have been reported. These age-related changes may not be physiologically significant.
PULMONARY FUNCTION TESTS
Lung function and exercise capacity decline with age in concert with numerous other physiologic, morphologic, and biochemical changes. Descriptions of “normal” age-related changes are confounded by an increasing prevalence of disease, chronic illness, medication use, and an increasingly sedentary lifestyle. Further, chronologic age only approximates physiologic age; the two often differ significantly. Chronologic age is, therefore, an imperfect measure for indexing changes with senescence. While it would be desirable to isolate the effects of biologic aging (aging in the absence of disease), it is essentially impossible to do so. The best studies to do so are longitudinal, tracing change with time, because they avoid the obvious biases of cross-sectional studies. Longitudinal studies, however, have methodologic problems and biases of their own, the most obvious being that the healthy elderly represent a healthy survival population. If, as a group, they have better than average lung function, they would not represent the general population of elderly people well.
LUNG VOLUMES
Figure 19-8 illustrates typical lung volume changes with aging based on cross-sectional studies. With the exception of vital capacity, the effect of aging on lung volumes is based on cross-sectional rather than longitudinal data because there are almost no longitudinal studies of static lung volumes. Total lung capacity (TLC), the volume of air in the lungs at the end of a maximal inspiration, is marked by the point at which the recoil pressure exerted by the respiratory system is exactly counterbalanced by the maximal inspiratory pressure generated by the respiratory muscles. Since both the compliance of respiratory system (lung and chest wall combined) and maximum inspiratory pressure fall with aging, TLC might also be expected to fall. However, in seven cross-sectional studies of TLC summarized by the European Coal and Steel Community, four of the studies in men and three of those in women did not find a significant age coefficient.35 The remaining studies found only small declines in TLC with age, on the order of –8 to –19 mL/yr. When these study results were combined into average equations, no significant age coefficients were reported for either men or women. McClaran et al. measured lung volumes twice in 18 healthy, fit men.36 The first measurement was at a mean age of 67 and the second was 6 years later. Although average TLC fell 25 mL/yr, the change was not statistically significant. The study was small and the interval was short.
Figure 19-8 Schematic illustration of lung volume changes with age based on cross-sectional studies in seated individuals. (TLC, total lung capacity; FRC, functional residual capacity; RV, residual volume; CC, closing capacity.) Although not labeled, vital capacity (VC) is TLC minus RV. The most consistent changes are an increase in RV and a decrease in VC. (Reproduced with permission from Peterson DD, Fish-man AP. Aging of the respiratory system, in Fishman AP (ed). Update: Pulmonary Diseases and Disorders. New York, McGraw-Hill, 1992, pp 1–17.)
In summary, current cross-sectional studies suggest that TLC either does not decline with age or declines very slowly. It is interesting to speculate on the possibility that cross-sectional studies of TLC might be confounded because they typically index TLC to both age and height. Height declines with aging, and maximum height during a life span appears to increase with successive generations. The author believes that longitudinal studies of TLC with age are likely to show small but significant declines with age.
Both slow and forced vital capacity (FVC) decline with age, more rapidly in men than women. Average decrements in vital capacity per year vary considerably; in cross-sectional studies, declines range from 21 to 33 mL/yr in men and 18 to 29 mL/yr in women. Theoretically, longitudinal studies should provide better estimates of the effect of aging on lung function. Ware et al., in a study containing both longitudinal and cross-sectional computations, found cross-sectional falls in FVC for men and women to be –34 and –27.8 mL/yr, respectively.37 The longitudinal estimates were –40 mL and –31.3 mL/yr, respectively. This study contradicts the generally held concept that longitudinal studies show smaller declines in FVC than cross-sectional studies. Currently, it is not certain whether longitudinal studies are all that much different from cross-sectional studies in describing declines in FVC and forced expiratory volume in 1s (FEV1). Longitudinal studies tend to show an acceleration in the rate of loss in FVC and FEV1 as age advances.
Cross-sectional studies of residual volume (RV) and the RV/TLC ratio consistently show increases with age. In the young, RV, the volume of air in the lungs at the end of a maximal expiration, is the volume at which the outward static recoil pressure of the respiratory system is counterbalanced by the maximal pressure exerted by the expiratory muscles. In older subjects, expiratory flow never completely reaches zero and the determination of RV is made partly by the length of time an individual can maintain expiratory effort.38 Other factors leading to an increased RV with aging include loss of lung recoil, decreased chest wall compliance, decreased expiratory muscle force, and increased small airway closure (air trapping) in dependent lung zones. Time of exhalation and increase in air trapping are probably more important than changes in lung and chest wall compliance in explaining the increase in RV with aging.
FRC is also determined by the balance of the elastic recoil forces of the lung and chest wall; but, in this instance, the equilibrium occurs at the end of a quiet (unforced) exhalation. Since lung recoil falls and the chest wall stiffens with age, one would expect FRC to increase. Cross-sectional studies, however, show inconsistent results, with most showing no change in FRC with aging. Studies that find an increase in FRC with aging show a small positive age coefficient on the order of 7 to 16 mL/yr. McClaran’s longitudinal study found FRC to increase 40 mL/yr, but again, the change was not significant.36 Despite the conflicting data, it is generally believed that FRC increases with aging.
Loss of lung recoil also changes the volume at which airway closure occurs. When adults exhale fully, small airways close in the region of the terminal bronchioles in dependent lung zones. The lung volume at which this closure begins is measured as closing volume or, if it is added to RV, closing capacity. Closing volume increases linearly with age from about 5% to 10% of TLC at age 20 to about 30% of TLC at age 70.39 The loss of lung elastic recoil, a possible decrease in the recoil of the intrapulmonic airways, and decreases in small airway diameter probably explain most of the changes in CV.
Closing volume encroaches on tidal volume by about age 44 when subjects are supine and about age 65 when they are seated (Fig. 19-8). Airway closure during tidal breathing explains most of the decrease in arterial oxygen tension (PAO2) observed with aging40,41 and may contribute to an aging-related increased frequency dependency of compliance.
AIRFLOW
While essentially all expiratory flows measured during a maximum expiratory maneuver decrease with age, the declines are most evident at lower lung volumes (Fig. 19-9). Nunn and Gregg, in a study of 225 male and 228 healthy female nonsmokers,42 reported a modest, nonlinear decrease in peak expiratory flow (PEF) with aging (Fig. 19-10), which reached a high point at age 30 to 35; a decline became evident at about age 45. After age 50, the average decline was about 4 L/min per year in men, about 2.5 L/min per year in women.
Figure 19-9 Illustrative maximal flow–volume curves for healthy “elderly” women (mean age, 63 years) and healthy young women (mean age, 25 years). Although all flows tend to be reduced with aging, the reduction in flow is most evident at lower lung volumes, where the flow–volume curve is clearly concave to the volume axis. (Reproduced with permission from Peterson DD, Fish-man AP. Aging of the respiratory system, in Fishman AP (ed). Update: Pulmonary Diseases and Disorders. New York, McGraw-Hill; 1992:1–18.)
Figure 19-10 Changes in peak expiratory flow in 225 males and 228 females who were healthy nonsmokers. The center line is a regression curve representing mean data and the boundaries are 90% confidence intervals. (Reproduced with permission from Nunn AJ, Gregg I. New regression equations for predicting peak expiratory flow in adults. Br Med J. 1989;298(6680):1068–1070.)
Figure 19-11, from Paoletti et al.,43 illustrates changes in FEV1 during growth, maturation, and senescence. Changes in FVC are similar. In one model of aging, FVC and FEV1 increase progressively during the growth phase until about age 12. In the maturation phase (during adolescence), there is an acceleration of these increases. Increases in FVC and FEV1 are seen up to about 20 years in women and about 25 years in men; increases in lung volumes occur even after somatic growth ceases. There appears to be a plateau phase where there is little or no change in FVC or FEV1 prior to the onset of a decline. However, Robbins et al. demonstrated that, while the plateau correctly represents average data, lung function is often increasing or decreasing in individuals (Fig. 19-12). Their study confirms the suspicion that the “plateau” phase represents the merging of slower maturation-related increases in FVC and FEV1 in some subjects, with subtle decreases in others.44 In the decline phase, there appears to be acceleration in the rate of loss of FVC and FEV1 as age progresses. An accelerated rate loss at older ages is, however, not found in all studies. The rate of decline in FVC and FEV1 with age tends to be greater: (1) in men; (2) in taller individuals; (3) in individuals with larger baseline values; and (4) in individuals with increased airway reactivity.
Figure 19-11 Change in FEV1 with age from a cross-sectional study of 538 females and 263 males selected as “normal” from a larger study of 3289 subjects. Changes in FVC are similar. (Reproduced with permission from Paoletti P, Pistelli G, Fazzi P, et al. Reference values for vital capacity and flow-volume curves from a general population study. Bull Eur Physiopathol Respir. 1986;22(5):451–459.)
Figure 19-12 Predicted FEV1 trajectories from 44 men based on linear regressions of longitudinal data. Nonsmokers (fine lines) and smokers (dashed lines). The heavy line is based on the entire group’s data. While the group’s data show no change with age, data for individuals show both increases and declines with age during this time period, when a plateau in lung function was theorized to occur. (Reproduced with permission from Robbins DR, Enright PL, Sherrill DL. Lung function development in young adults: Is there a plateau phase? Eur Respir J. 1995;8(5):768–772.)
AIRWAYS RESISTANCE
Total airway resistance measured at FRC does not change with aging. Since upper airways increase and smaller airways decrease in size with aging, it is likely that peripheral airway resistance increases and central airway resistance decreases. That total airway resistance does not change with aging may be a function of the counterbalancing of these two opposite changes. However, since about 90% of total airway resistance resides in the upper airways, significant changes in peripheral airway resistance might not be readily reflected in total airway resistance. Significant increases in peripheral airway resistance with age also would be consistent with the more dramatic decreases in maximum flow observed at low lung volumes.
GAS EXCHANGE
The carbon monoxide diffusing capacity (DLCO), also known as transfer factor (TLCO), declines with age. Earlier cross-sectional studies report a linear decline in DLCO of about –0.2 mL CO/min per mm Hg per year for men and –0.15 mL CO/min per mm Hg per year for women. These declines are roughly 0.5% per year. In a large representative sample of US adult men, Neas and Schwartz found an almost linear fall in DLCO.45 In women, however, they found a nonlinear, quadratic decline in DLCO with age. After age 47, the nonlinear component was not significant and the decline in DLCO was identical to that in the earlier studies. The decline in DLCO with age did not vary with race.
The decline in DLCO with age is not explained by increased nonhomogeneity of gas distribution. Measured DLCO falls as alveolar PO2 increases, and venous hemoglobin concentration falls. Neither alveolar PO2 nor hemoglobin concentration varies enough with age to explain the aging decline in DLCO. The magnitude of the decline in DLCO corresponds fairly well to the magnitude of the known aging-related decrease in the internal surface area of the lung.
The components of DLCO are membrane diffusing capacity (Dm) and pulmonary capillary blood volume (Vc). Both Dm and Vc decrease with age. In a cross-sectional reference value study of 54 male and 36 female healthy nonsmokers, the decline in Dm and Vc with age were found to be linear.46 Membrane diffusing capacity fell at about 0.6% per year in both men and women. Pulmonary capillary blood volume fell at about 0.3% per year.
Although alveolar oxygen pressure (PAO2) remains constant with age, arterial PO2 (PaO2) decreases and the alveolar–arterial oxygen tension gradient (PA – aO2 increases with aging (Fig. 19-13).47 The decline in PAO2 with aging is more pronounced when subjects are studied in a recumbent as contrasted with an upright position. The most likely explanation for the decline in PAO2 with aging is increased mismatching of ventilation to blood flow (E/
) as airway closure begins to occur during tidal breathing. Increased (
E/
) mismatching with aging is also associated with an increase in physiologic dead space.48,49 Hypoventilation does not contribute to the age-related fall in PaO2, since PaCO2 and pH do not change with age (Fig. 19-13).
Figure 19-13 Change in PaO2, PaCO2 and A-a gradient P(A-a)O2 with age. Data were obtained from 200 healthy men and women living in Salt Lake City, UT (altitude = 1400 m). Sea-level data would be similar, with a small upward shift in PaO2 and PaCO2.
EXERCISE CAPACITY
Peak (
peak) and maximum work capacity decrease with aging in both sedentary and active individuals.50
peak (L/min) increases until about age 20. Declines are evident at about age 25 in both men and women and continue at about 1% per year (Fig. 19-14). If one expresses
peak as a function of body weight (L/kg per min), the decline is evident much earlier, perhaps in the first decade of life. The magnitude of the decline in
peak tends to be greater in longitudinal than in cross-sectional studies and occurs roughly twice as fast in sedentary than in physically active persons. Most, but not all, studies report linear declines in
peak with age, even though a nonlinear decline would be expected based on the number and type of variables that affect exercise capacity.
Figure 19-14 Decline in maximum exercise capacity with age. Exercise capacity declines nonlinearly with age. Maximum work capacity correlates strongly with peak oxygen uptake. (Reproduced with permission from Jones NL, Summers E, Killian KG. Influence of age and stature on exercise capacity during incremental cycle ergometry in men and women. Am Rev Respir Dis. 1989;140(5):1373–1380.)
The decline in exercise capacity with age occurs as a result of normal aging but is accelerated by lifestyle issues. Aging is associated with significant changes in body configuration. Specifically, there is an increase in total body weight, primarily representing an increase in fat mass, since fat-free mass (mostly muscle mass) decreases with aging. The changes are most pronounced in sedentary persons. Muscle mass decreases, with a preferential atrophy of type II muscle fibers, and is associated with a decrease in muscle capillarization and oxidative activity. Muscle strength decreases on the order of 2%/yr from ages 20 to 70. Variables associated with loss of exercise capacity with aging are listed in Table 19-2.
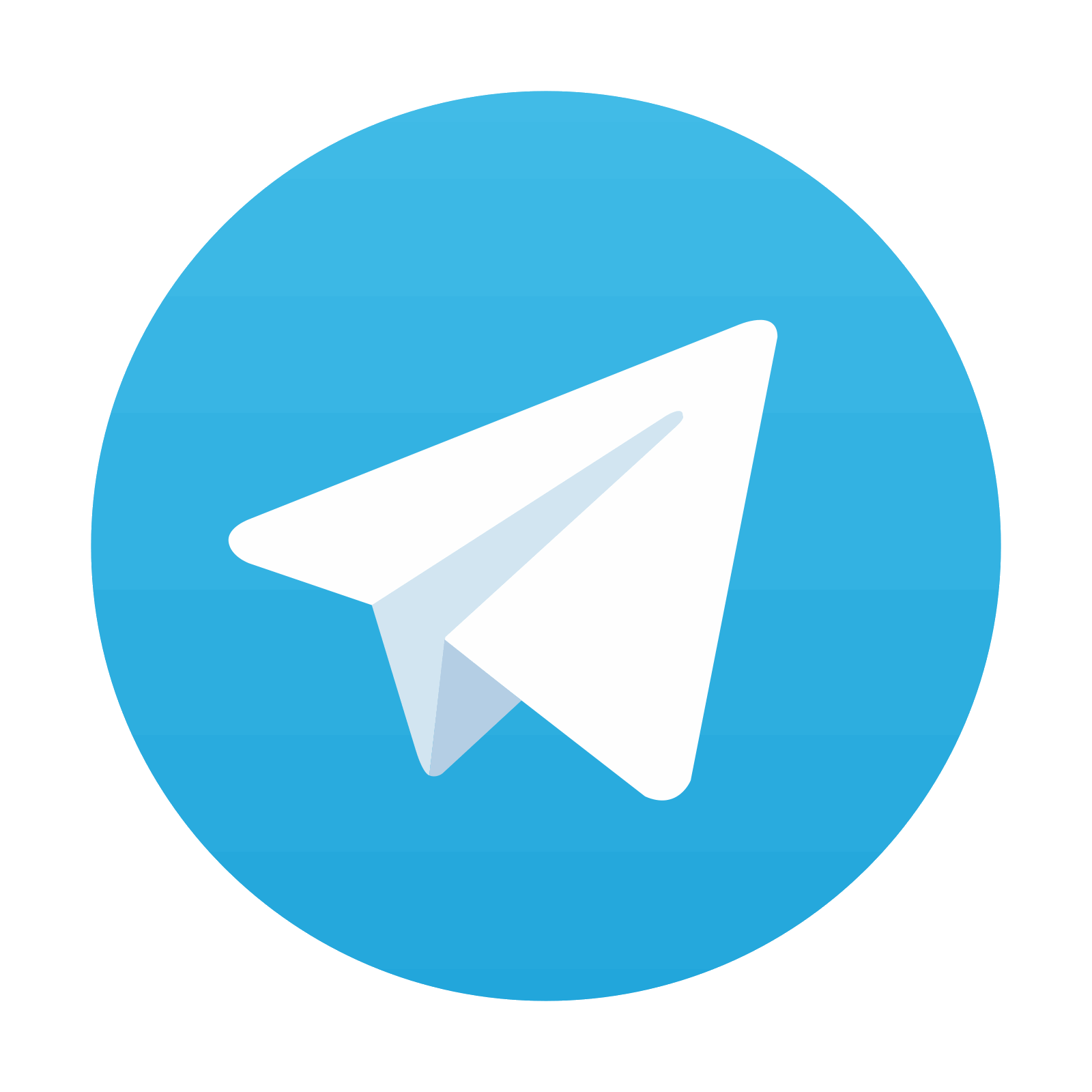
Stay updated, free articles. Join our Telegram channel
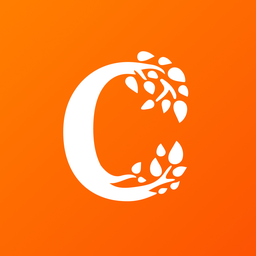
Full access? Get Clinical Tree
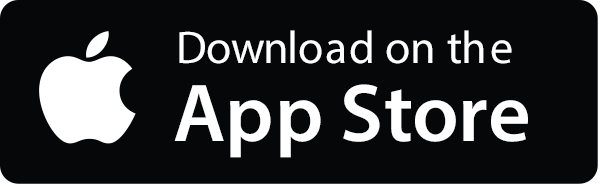
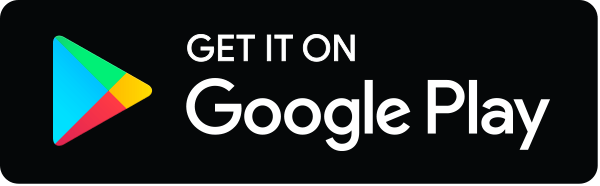