Maria Drakopoulou1, 2, 3, Konstantinos Dimopoulos1, 2, Stamatia Prapa1, 2, Darryl F. Shore1, 2, Stella Brili3, and Michael Gatzoulis1, 2 1Adult Congenital Heart Centre, Royal Brompton Hospital, London, UK 2National Heart and Lung Institute, Imperial College London, London, UK 3Hippokration Hospital, Athens, Greece The successful management and correction of congenital heart defects during infancy have resulted in an increasing number of adults with congenital heart disease (CHD). Due to medical and surgical advances, 85% of children born with CHD now survive to adulthood [1–4]. It is imperative that this group of young adults is not lost to follow‐up [5]. Smooth transition of these individuals from pediatric to adult care is an essential but often difficult process for both the clinician and the patient. Important issues such as residual hemodynamic lesions, arrhythmia management, endocarditis prophylaxis, career, and family planning should be addressed in an appropriate and timely fashion [6]. In fact, as this population ages, long‐term complications become more frequent, posing a significant burden and challenge to healthcare providers [4, 7]. Adults with CHD may be divided into those with previous repair or palliation and those with unrepaired lesions. While patients with repaired lesions are more likely to have had relief of the hemodynamic burden posed by the original cardiac defect, residual hemodynamic lesions and sequelae from surgery, such as scar‐related arrhythmias, are frequent. Follow‐up is thus essential in all, even the most stable adult CHD (ACHD) patients. Patients with unrepaired lesions are those with a delayed or missed diagnosis and those in whom repair was impossible or considered too high risk at the time. Those with persistent unrepaired lesions may at times undergo palliative procedures as a definitive approach or a bridge to repair, even though the latter is rare in adult life. Certain lesions may be amenable to late repair (e.g., atrial septal defects, ASDs), but long‐term complications are frequent even after successful repair. In specialist ACHD centers, approximately one‐half of operations are first repairs, while the other half are reoperations following reparative or palliative procedures [8]. The number of operations on ACHD patients has increased dramatically in recent years and reflects the growing number of patients and the highly complex physiology and anatomy of patients with CHD reaching adulthood [8]. There are numerous issues to be considered when caring for ACHD patients, many of which are unique to this population. Uncorrected lesions can lead to volume overload, heart failure, arrhythmias, or chronic cyanosis. Previously repaired or palliated patients are likely to require reoperations with an inherently higher risk (e.g., repeat sternotomy, adhesions, thrombotic or air embolism related to retrosternal conduits). Finally, as this population ages, the risk of acquired heart disease (e.g., coronary artery disease, valvar disease) increases and its burden superimposes on the congenital defect, accelerating deterioration [4]. Heart failure management in the setting of CHD is challenging given the wide range of ages at which it occurs, the heterogeneity of the underlying anatomy and surgical repairs, the wide spectrum of causes, and the paucity of evidence demonstrating treatment efficacy [9]. The European Society of Cardiology Guidelines state the following definition for heart failure: Heart failure is a syndrome characterized by typical symptoms (e.g., breathlessness, ankle swelling and fatigue) that may be accompanied by signs (e.g., elevated jugular venous pressure, pulmonary crackles and peripheral oedema) caused by a structural and/or functional cardiac abnormality, resulting in reduced cardiac output and/or cardiac pressures at rest or during stress. [10] Acute heart failure refers to the rapid onset or worsening of symptoms or signs of heart failure, whereas chronic cardiac decompensation is considered as a persistent syndrome that requires consideration of therapy to prevent progression, decompensation, and death [11]. The clinical presentation of heart failure in patients with ACHD may vary significantly by defect or age [9, 12]. Patients with ACHD can have classic symptoms of fatigue, dyspnea, and exercise intolerance, but may also manifest more subtle signs of malnutrition, growth failure, or cachexia [9]. Symptoms of heart failure in ACHD patients may result from a variety of mechanisms, both cardiac and extracardiac. A persistent or residual defect or hemodynamic lesion can result in chronic volume or pressure overload and ventricular dysfunction. Coronary anomalies, pericardial disease, arrhythmias, chronotropic incompetence, and myocardial injury during surgery may also affect cardiac function and output. Extracardiac factors include pulmonary parenchymal and vascular disease, cyanosis and pulmonary arterial hypertension, skeletal abnormalities, and detraining. Patients with CHD often adapt to their long‐standing limitations; therefore, they may not report symptoms despite significant objective exercise impairment [13]. Subjective evaluation of exercise intolerance using general heart failure classifications such as the New York Heart Association (NYHA) categories, the modified Ross, or the Warnes–Somerville classification may underestimate the severity of the disease, particularly in patients with complex or cyanotic CHD [13]. In fact, asymptomatic ACHD patients often have abnormal exercise capacity and a high prevalence of asymptomatic ventricular dysfunction [14, 15]. Most importantly, none of the available heart failure classification grading scales has been validated on predicting outcomes. Therefore, objective assessment of exercise capacity by cardiopulmonary exercise testing is advocated for all patients with CHD, particularly those for whom management may be influenced by results or who would benefit from understanding objective limitations to exercise [16]. Patients with an objective reduction in exercise tolerance may be considered for earlier pharmacologic or hemodynamic intervention that may improve myocardial performance and exercise capacity, regardless of symptoms. Cardiopulmonary exercise testing with the use of an incremental treadmill adjusted to the level of exercise limitation appears ideally suited for assessing patients with ACHD. Assessment of peak oxygen consumption (VO2), heart rate and blood pressure response, oxygen pulse, and the ventilatory response to exercise provides invaluable information about the severity and mechanisms of exercise intolerance. A gradual decline in the percentage of predicted VO2 has been observed across the spectrum of patients with CHD (Figure 47.1) [17]. Baseline assessment can identify early deterioration if repeated when symptoms develop or at intervals in the absence of overt symptoms. Cardiopulmonary exercise testing also provides strong prognostic information in adult patients with CHD. Interestingly, the combination of peak VO2 and heart rate reserve provided the greatest predictive information after adjustment for clinical parameters [17]. Prior to intervention, cardiopulmonary exercise testing may also predict surgical outcome [18]. During follow‐up, it may be used to assess the effect of intervention. At the onset of symptoms, patients with CHD and heart failure should undergo right‐ and left‐sided anatomic and hemodynamic evaluation for reversible or repairable structural abnormalities that may contribute to symptoms. These could be valve lesions (e.g., pulmonary valve regurgitation after repair of tetralogy of Fallot [TOF] or tricuspid regurgitation in patients with congenitally corrected [L‐] transposition of the great arteries) or other obstructive lesions (e.g., baffle stenosis in patients with previous Mustard or Senning repair) [19]. Cardiac imaging by echocardiography and cardiovascular magnetic resonance are a fundamental part of the preoperative assessment, perioperative management, and long‐term follow‐up. In particular, cardiovascular magnetic resonance imaging (MRI) is considered ideal for quantifying right ventricular function, which is commonly affected by ACHD [16]. Patients with CHD may develop pulmonary arterial hypertension, which can present as heart failure. A thorough workup, including cardiac catheterization with reversibility challenge testing, should be considered to evaluate the cause of pulmonary arterial hypertension, its severity, and eligibility for targeted advanced therapies. When managing an ACHD patient with signs and symptoms of heart failure, certain guidelines are reasonably presumed to be beneficial, such as the application of existing heart failure guidelines for tobacco cessation, weight management, and routine screening and management of cardiovascular risk factors. However, other guidelines should be extrapolated, with the recognition that data are sparse and inconclusive for patients with ACHD. While there is mounting evidence to suggest common pathophysiologic mechanisms between acquired heart failure and ACHD, including neurohormonal activation, established heart failure therapies counteracting neurohormonal activation are not widely used in ACHD. This is due to the lack of evidence supporting the efficacy and safety of these therapies in ACHD, which has prevented the establishment of evidence‐based guidelines for the pharmacologic treatment of these patients. Figure 47.1 Distribution of percentage predicted peak oxygen consumption (peak VO2) in different diagnostic groups. AVSD, atrioventricular septal defect; med, median; q25 and q75, 25th and 75th percentiles; RV, right ventricle; syn, syndrome; TOF, tetralogy of Fallot. Source: Inuzuka et al. 2012 / With permission of Wolters Kluwer Health, Inc. Few studies have examined the effect of heart failure medications in patients with ACHD, all of which were limited by a small sample size [12, 20]. Since few pharmacologic data exist in this patient population, clinicians should exercise caution if they extrapolate results from pharmacologic trials that were performed in patients with acquired heart disease and apply those results to patients with CHD. This approach is arbitrary and ignores important differences between the two conditions and the unique characteristics of the individual with CHD that could affect dosage, tolerance, and effectiveness [21]. The use of the same therapies would, by extension, be beneficial in selected patients with biventricular circulation and evidence of systemic systolic heart failure. Less benefit may be expected in patients with biventricular circulation with a systemic right ventricle (RV) and systolic dysfunction, or those with single‐ventricle physiology palliated with a Fontan repair. Some physiologic circumstances may pose a particularly high risk of adverse effects of systemic vasodilators, including angiotensin‐converting enzyme inhibitor, angiotensin receptor blockade, or hydralazine. Thus, these agents should be used cautiously, with an understanding of the patient’s unique physiology and serial evaluation for potential adverse effects. Cardiac resynchronization is a new therapeutic approach. Benefit has been reported in patients with severe left ventricular dysfunction associated with TOF and it may be considered in select patients [22]. Application of device therapy in patients with CHD imparts unique challenges. Venous access can be difficult for both initial implantation and especially for follow‐up lead replacement, because patients had previous cardiac surgeries and they also need device therapy for extended periods of time. Before transvenous implantation, patients should be evaluated for venous stenosis and residual shunting. Identified shunts should be closed if there is more than a twofold increase in systemic thromboembolic risk. For patients in whom there is limited or no venous access, an epicardial approach can be used but requires a limited thoracotomy or sternotomy. Given the benefit of MRI in patients with CHD, consideration should be given to the use of MRI‐compatible devices when possible. Finally, heart transplantation remains a surgical procedure of choice for eligible patients with severe advanced heart failure that persists after maximal medical, surgical, and arrhythmia treatment [23]. The body of information related to transplantation for CHD is derived almost entirely from registry and single‐center–based outcome data; no randomized, clinical trial, or meta‐analysis data are available. Adult patients with CHD represent an increasing proportion of heart transplant recipients. Adults with end‐stage CHD have unique pathophysiologic and comorbidities requiring tertiary care. As the ACHD population ages and increases in number, randomized controlled trials are urgently needed to rationalize treatment of heart failure in these patients. Despite advances in cardiac surgery that have allowed repair of CHD at an early age, it is estimated that approximately 5–10% of patients with CHD develop pulmonary arterial hypertension [24, 25]. The diagnosis of pulmonary arterial hypertension requires a comprehensive set of investigations to confirm that hemodynamic criteria are met and to find the etiology and severity of the condition. Pulmonary arterial hypertension is defined as an increase in mean pulmonary arterial pressure (PAPm) ≥25 mmHg at rest. The current definition relies on the presence of mean pulmonary arterial pressure exceeding 25 mmHg at rest as assessed by right heart catheterization, a pulmonary artery wedge pressure below 15 mmHg, and resting pulmonary vascular resistance (PVR) >3 Wood units. The diagnosis of pulmonary vascular disease itself requires special attention and expertise in pulmonary arterial hypertension–CHD for management guidance. In all patients with shunts, accurate estimation of pulmonary (Qp) and systemic blood flow (Qs) is important not only for calculating PVR, but also for quantifying the magnitude of the shunt and for deciding whether the patient is a good candidate for surgery. Pulmonary arterial hypertension associated with adult CHD is included in group 1 of the pulmonary hypertension clinical classification and represents a very heterogeneous patient population [26]. A specific anatomic–pathophysiologic classification is provided to better characterize each individual patient with pulmonary arterial hypertension associated with adult CHD. According to this classification, congenital systemic to pulmonary shunts associated with pulmonary arterial hypertension can be divided into (i) simple pre‐tricuspid shunts, such as ASD, and unobstructed total or partial anomalous pulmonary venous return; (ii) simple post‐tricuspid shunts, such as ventricular septal defect (VSD) and patent arterial duct; (iii) combined shunts; and (iv) complex shunts such as common arterial trunk, single ventricle with unprotected pulmonary circulation, and atrioventricular septal defects (AVSD). VSDs are the most frequent simple defects causing pulmonary arterial hypertension, with an estimated 10% of all VSDs and 50% of large VSDs having the potential to cause Eisenmenger syndrome if not repaired by 2 years of age [27]. Recent pulmonary hypertension guidelines have recognized the wide spectrum of pulmonary arterial hypertension–CHD by suggesting a clinical classification into four major groups: (i) Eisenmenger syndrome; (ii) pulmonary arterial hypertension associated with systemic to pulmonary shunts; (iii) pulmonary arterial hypertension with coincidental small defects; and (iv) pulmonary arterial hypertension after corrective cardiac surgery [26]. There are two additional categories of pulmonary vascular disease that are related to CHD: (i) segmental pulmonary arterial hypertension; and (ii) raised PVR in Fontan patients. Each of these groups has distinct pathophysiologic features and thus differs in its management and overall outcome. Persistent exposure of the pulmonary vasculature to increased blood flow due to systemic to pulmonary shunts, as well as increased pressure, may result in a typical pulmonary obstructive arteriopathy (identical to other pulmonary arterial hypertension forms) leading to an increase in PVR. Eisenmenger syndrome is the extreme manifestation of the spectrum of pulmonary arterial hypertension in association with CHD. It includes patients with large left‐to‐right shunts, which result in significant pulmonary arterial hypertension (systemic or near‐systemic levels) and subsequent reversal of the shunt, which may become right‐to‐left or bidirectional, and ultimately lead to cyanosis. Currently up to 4% of ACHD patients followed in tertiary centers have Eisenmenger syndrome, as opposed to 8% in previous eras when early diagnosis and repair were not available [28]. This number is expected to fall further. The clinical manifestations of pulmonary arterial hypertension secondary to CHD can vary depending on the underlying heart defect, patient age, repair status, and degree and direction of shunting. The symptoms are nonspecific and mainly related to progressive right ventricular dysfunction. Initial symptoms are typically induced by exertion. Common nonspecific symptoms include shortness of breath, fatigue, weakness, angina, and syncope. Less commonly, patients may also describe dry cough and exercise‐induced nausea and vomiting [28]. Exercise intolerance is the cardinal symptom of pulmonary arterial hypertension. Patients with significant pulmonary arterial hypertension have greatly reduced exercise tolerance, which affects their functional status and quality of life [29]. In particular, patients with Eisenmenger syndrome have been found to have the lowest exercise capacity of all ACHD patients, followed by patients with complex cardiac anatomy and univentricular circulation. This is attributable to a combination of pulmonary arterial hypertension and cyanosis, both of which contribute to a significant increase in physiologic dead space. In some patients, the clinical presentation may be related to mechanical complications of pulmonary arterial hypertension and the abnormal distribution of blood flow in the pulmonary vascular bed. These include hemoptysis related to rupture of hypertrophied bronchial arteries, as well as symptoms attributable to pulmonary arterial dilatation such as hoarseness caused by compression of the left recurrent laryngeal nerve, wheeze caused by large airway compression, and angina due to myocardial ischemia caused by compression of the left main coronary artery. Significant dilatation of the pulmonary artery may result in its rupture or dissection, leading to signs and symptoms of cardiac tamponade. The physical signs of pulmonary arterial hypertension include left parasternal lift, an accentuated pulmonary component of the second heart sound, a right ventricular third heart sound, a pansystolic murmur of tricuspid regurgitation, and a diastolic murmur of pulmonary regurgitation. Elevated jugular venous pressure, hepatomegaly, ascites, peripheral edema, and cool extremities characterize patients with advanced disease. Wheeze and crackles are usually absent. Eisenmenger syndrome, the most severe form of pulmonary arterial hypertension, is a multisystem disorder. The signs and symptoms of Eisenmenger syndrome result from pulmonary hypertension, low arterial O2 saturation, and hematologic changes, including secondary erythrocytosis, thrombocytopenia, and at times leucopenia. They also include dyspnea, fatigue, and syncope. Patients with Eisenmenger syndrome also present with hemoptysis, cerebrovascular accidents, brain abscesses, coagulation abnormalities, and sudden death. Moreover, patients with Eisenmenger syndrome have a high prevalence of peripheral organ dysfunction such as renal disease and hepatic dysfunction as a result of long‐standing hypoxia, on which we will expand later in this chapter. Patients with Eisenmenger syndrome have reduced life expectancy, although many survive into their third or fourth decade, with some living to their seventh decade [30–36]. A multivariable mortality risk stratification model based on five simple, noninvasive predictors of death (age, pre‐tricuspid shunt, presence of sinus rhythm, oxygen saturation at rest, presence of pericardial effusion) has been recently proposed by Kempny and colleagues [37]. Routine evaluation of patients with pulmonary arterial hypertension associated with CHD should include a chest radiograph, electrocardiogram (ECG), measurement of arterial oxygen saturations at rest and during exercise, formal exercise testing, and echocardiography. Cardiac catheterization is often necessary to establish the diagnosis and severity of pulmonary arterial hypertension, as well as to assess pulmonary vasoreactivity. Routine laboratory testing should focus on hemoglobin concentration, platelet count, and the detection of iron deficiency. Finally, cardiovascular magnetic resonance (CMR) imaging and high‐resolution chest computed tomography (CT) may provide additional information on right ventricular size and function as well as on the pulmonary vascular bed [28, 38, 39]. The management of patients with pulmonary arterial hypertension associated with CHD, and in particular those with Eisenmenger syndrome, is mainly based on the clinical experience of experts rather than being formally evidence based [40–43]. The general care of patients with pulmonary arterial hypertension consists of preservation of fluid balance, management of secondary erythrocytosis, appropriate iron supplementation, and abolition of routine venesections [44]. Anticoagulation may be indicated, especially for patients with documented pulmonary thrombosis and embolic phenomena, in the absence of prior severe hemoptysis. An operation may be considered in patients with prevalent systemic to pulmonary shunting [41]. The diagnosis of pulmonary vascular disease requires special attention and expertise in ACHD. The criteria for shunt closure are based on baseline PVR index and have been proposed in recent pulmonary hypertension guidelines based on available literature data [26]. When a large left‐to‐right shunt is present, accurate estimation of PVR index becomes essential, as the increase in pulmonary blood flow due to the shunt may cause a significant rise in pulmonary arterial pressure with little or no rise in PVR (Figure 47.2) [41]. Patients with PVR index <4 Wood units m2 may be considered for repair (with surgery and/or percutaneous procedure), whereas patients with PVR index <8 Wood units/m2 should not be considered for repair. Patients with PVR index 4–8 Wood units/m2 should be individually evaluated in tertiary centers. Additional criteria include the type of defect, age, pulmonary vascular resistance : systemic vascular resistance ratio, and Qp : Qs ratio. No prospective data are available on the contribution of vasoreactivity testing, closure test, or lung biopsy for operative assessment. Surgical or percutaneous intervention is contraindicated in patients with Eisenmenger syndrome and likely not necessary in patients with small/coincidental defects. Recently, new pharmacologic options became available for patients with pulmonary arterial hypertension, including those with CHD [28, 45]. Based on the results of BREATHE 5 (the only randomized trial in this cohort) and other nonrandomized trials, advanced pulmonary hypertension therapy is currently indicated for highly symptomatic patients with Eisenmenger syndrome (NYHA functional class III or IV) [45–48]. Figure 47.2 The importance of calculating pulmonary vascular resistance in patients with congenital heart disease. In this plot, the formula for indexed pulmonary vascular resistance (PVRi) is used to graphically represent changes in mean pulmonary arterial (PA) pressure with increasing pulmonary blood flow, for various levels of PVRi. LA, left atrial; PAH, pulmonary artery hypertension; PVD, pulmonary vascular disease. Source: Dimopoulos et al. 2014 / With permission of Oxford University Press. Enhanced recovery after surgery implementation and PDE‐5s sildenafil and tadalafil therapy have shown favorable functional and hemodynamic results in patients with pulmonary arterial hypertension associated with CHD and Eisenmenger syndrome [40, 41, 49, 50]. The use of intravenous epoprostenol has been reported in patients with Eisenmenger syndrome, with favorable effects on hemodynamics and exercise capacity, although central lines expose patients to the risk of paradoxical embolism and sepsis. No data are available on the use of other prostanoids. Advanced pulmonary arterial hypertension therapies have also been used to reduce the risk of developing postoperative pulmonary arterial hypertension and for treating this in patients undergoing surgical repair of CHD or acquired valve disease [39, 51]. Whether such therapies could be used to treat and subsequently repair congenital defects that have already led to the development of pulmonary hypertension remains unclear. In fact, the long‐term efficacy and safety of advanced therapies in pulmonary arterial hypertension related to CHD warrant further investigation. Moreover, available data on closure of intra‐ or extracardiac communications in the presence of severe pulmonary arterial hypertension, with or without the use of advanced therapies, are scarce and limited to case reports [39]. Currently, reparative surgery is indicated only in patients with evidence of pulmonary arterial reactivity and/or at least 1.5 : 1 left‐to‐right shunting. Arrhythmias in patients with ACHD are the most frequent reason for hospital admission and a prominent cause of morbidity and mortality. Arrhythmias are the result of sudden hemodynamic changes, or more commonly reflect the chronic interplay between the cardiac defects, hemodynamic lesions, and postoperative scarring (Table 47.1) [11]. The entire spectrum of rhythm abnormalities can be encountered in adults with CHD. Table 47.1 Rhythm disturbances in adults with congenital heart disease. Source: Warnes CA et al. 2008 / With permission of Elsevier. Supraventricular arrhythmias are the most commonly encountered arrhythmias in ACHD patients. It has been estimated that approximately 50% of 20‐year‐old patients with CHD will develop an atrial tachyarrhythmia during their lifetime. Supraventricular arrhythmias are more frequent in patients with atrial dilatation (e.g., atriopulmonary Fontan) and those with a history of surgery involving extensive atrial incisions (e.g., Mustard, Senning, or Fontan procedure). Among supraventricular arrhythmias, intraatrial reentry tachycardia is common among ACHD patients and is initiated and sustained by viable myocardial fibers embedded within areas of scar tissue [52, 53]. Atrial fibrillation is also common in ACHD, especially in patients with significant atrial dilatation due to unrepaired lesions or residual hemodynamic burden. Ventricular arrhythmias are believed to be the leading cause of sudden death in several subtypes of CHD, with an overall risk that is up to 100‐fold higher than in age‐matched controls. Fortunately, the absolute incidence of these devastating events remains relatively low, at approximately 0.1% per year [54]. Macroreentrant circuits are also responsible for ventricular arrhythmias and are encountered in patients with repaired TOF. In this case, the circuit involves the isthmus between the outflow tract patch and the tricuspid annulus [53]. Bradyarrhythmias are also commonly encountered in patients with ACHD, and their incidence and timing depend on the type of cardiac defect and/or previous palliation/repair. Bradyarrhythmias may involve disorders of the sinus node, atrioventricular (AV) node, His–Purkinje system, or intraatrial propagation. Sinus node dysfunction can be present at birth due to congenital abnormalities such as sinus venosus ASD and AV discordance with left atrial isomerism. It may also occur following cardiac surgery (Mustard, Senning, or Fontan procedure). Often, loss of sinus rhythm in postoperative cases is gradual, suggesting a chronic hemodynamic process rather than an occurrence of direct surgical injury of the sinus node [53]. An AV block can occur spontaneously, especially in congenital defects with conduction system abnormalities (e.g., AVSD and congenitally corrected transposition) or postoperatively. Surgery commonly associated with acquired AV block includes closure of VSD, relief of subaortic stenosis, replacement or repair of an AV valve, and TOF repair. Surgical manipulation of the ventricular septum may cause AV block because of either direct injury or secondary damage of the interventricular conduction system associated with an inflammatory response [55]. Conduction abnormalities can worsen functional capacity because bradycardia or atrioventricular dyssynchrony may lead to impaired ventricular function. In fact, junctional rhythm can lead to elevated atrial pressure in individuals palliated with a Fontan procedure for single‐ventricular physiology or those with restrictive physiology, which can be reversed by permanent pacing to restore atrioventricular synchrony. The clinical manifestations of arrhythmia in patients with CHD range from completely asymptomatic to rapid deterioration or sudden cardiac death. The onset of arrhythmia often heralds a hemodynamic decline and contributes to a vicious cycle of progressive clinical deterioration. Persistent atrial tachycardia may also lead to intracavity thrombus formation and thromboembolic events [56]. Most importantly, sudden cardiac death secondary to documented or presumed arrhythmia is the leading cause of mortality in adult patients with CHD, with a higher risk in patients with unrepaired cyanotic or left‐sided obstructive lesions [57]. The first and most effective step in the management of recurrent or persistent arrhythmias in ACHD is identification and correction of hemodynamic abnormalities [58]. The occurrence of sustained atrial or ventricular arrhythmias may be a cause or a consequence of a change in hemodynamic status and should prompt the clinician to look for reversible causes of arrhythmia, especially structural lesions that can be treated. Correction of hemodynamic lesions is usually accompanied, in our practice, by electrophysiologic mapping and ablation in the catheter laboratory, usually before surgery. Catheter ablation and surgical interventions are, in fact, increasingly used in ACHD for both supraventricular and ventricular arrhythmias. Today, new three‐dimensional mapping techniques and increasing expertise allow mapping and ablation of complex arrhythmogenic circuits. The rationale for preoperative ablation is that it reduces hemodynamic burden perioperatively, especially in the presence of persistent supraventricular tachycardia, by lowering the risk of hemodynamic instability during anesthesia induction. Moreover, preoperative targeted transcatheter ablation prevents the creation of unnecessary surgical scars that, in themselves, may provide the substrate for tachycardias. Therefore, our current practice is that supraventricular tachycardias should be treated by mapping and ablation in the catheter laboratory before surgery. If ablation fails to address the arrhythmia, surgical ablation becomes a viable alternative. Evaluation for arrhythmias should be performed at the onset or worsening of symptoms, including resting and ambulatory ECG monitoring, pacemaker interrogation, or other testing such as an electrophysiology study as deemed appropriate. Patients with episodes of documented or strongly suspected ventricular tachycardia (VT) should also undergo preoperative mapping and ablation if possible. Implantable cardioverter defibrillators (ICD) are also used in patients with CHD, despite a lack of solid evidence to support this approach. Risk stratification is essential for identifying patients at a high risk of sudden death who would benefit from implantation of an automatic ICD or invasive electrophysiologic studies. Several predictors of sudden cardiac death have been proposed for patients with TOF, including a prolonged QRS duration, presence of symptomatic dysrhythmias, and inducible VT on electrophysiologic study [57, 59, 60]. Currently, according to guidelines from the American College of Cardiology, the European Society of Cardiology, and the American Heart Association, ICDs are indicated for secondary prevention in patients with sustained VT or resuscitated cardiac arrest in the absence of a reversible cause [61]. Risk–benefit assessment for primary prevention ICDs is a major challenge. In our practice, patients with TOF who have poor left or right ventricular function, evidence of extensive scarring on CMR imaging, and prolonged QRS duration are referred for ICD implantation. While patients with preserved ventricular function, narrow QRS complex, and minimal scarring are not candidates for ICD implantation, there remains a group of patients who may be at risk of sudden death and for whom the indication for ICD implantation remains unclear. ICD indications for patients with systemic right ventricles or functionally univentricular hearts remain contentious. Challenges to ICD implantation include obstructed veins, conduits and baffles, atrioventricular valve disease, and intracardiac shunts. In selected patients, customized systems with epicardial and/or subcutaneous coils may represent a viable solution. Alternatively, the subcutaneous ICD is an attractive option for patients in whom transvenous access is not feasible or desirable and in whom bradycardia and antitachycardia pacing features are not essential. Continued advances in risk stratification and device technologies carry the potential to further improve efficacy and safety outcomes in this growing population of patients. Antiarrhythmic agents are often used in patients with ACHD, but most have proven to be disappointing, especially for the long term. Clinicians should exercise caution when selecting antiarrhythmic drug therapy for the patient with CHD because underlying bradyarrhythmias and ventricular dysfunction predispose these patients to proarrhythmic and negative inotropic consequences of drug therapy. Amiodarone and beta blockers are the most commonly used drugs, with amiodarone being the most effective. However, significant long‐term amiodarone toxicity makes ablative treatment even more important. Patients with atrial tachycardia will often require long‐term anticoagulation to prevent thrombus formation. This is especially true in patients with a Fontan circulation and those with intracardiac communications. However, anticoagulation may prove problematic in patients with Eisenmenger syndrome who are prone to hemoptysis and in patients with vascular malformations. Data on novel anticoagulants in CHD are scant, and not all patients with CHD will meet the indications for their use. Pacing for bradyarrhythmias can prove challenging in ACHD patients. Limited access to the heart due to congenital venous anomalies or acquired venous occlusion, surgical obstacles such as conduits and baffles, and the presence of intracardiac shunts with a significant thromboembolic risk may preclude endocardial lead placement and require an epicardial approach [62]. Cyanosis can be defined as bluish coloration of the skin and mucous membranes that is a consequence of an increased quantity of desaturated hemoglobin or derivatives of hemoglobin in the vessels of those districts [63]. Cyanosis is “peripheral” when it is secondary to slow peripheral flow of normally saturated blood, leading to increased oxygen extraction, and “central” when it is secondary to desaturation of arterial blood. Cyanosis in a patient with unrepaired or palliated complex CHD indicates the presence of a right‐to‐left shunt at the atrial, ventricular, or ductal level. Persistent right‐to‐left shunting through unrepaired CHD results in central cyanosis. Shunting leading to cyanosis may occur through intracardiac (ASD or VSD) or extracardiac (e.g., patent arterial duct) communications in the presence or absence of pulmonary hypertension (e.g., VSD, pulmonary stenosis). Cyanosis may also occur due to AV malformations within the lung or other organ segments. Long‐standing cyanosis is a multisystem disorder, causing multiorgan failure and a range of hematologic, neurologic, and metabolic disorders. Chronic hypoxia results in an increase in erythropoietin production and an isolated rise in red blood cell count (secondary erythrocytosis). This a physiologic adaptation phenomenon to chronic hypoxia aimed at improving oxygen transport. The term “polycythemia” is often inappropriately used to describe this phenomenon. However, polycythemia implies proliferation of all three cell lines (red and white blood cells and the platelets). In chronic cyanosis, there is no increase in white cell count and the platelet count is normal or, most often, decreased [63, 64]. Moreover, while polycythemic patients may benefit from regular venesections, this is not true for cyanotic ACHD patients with secondary erythrocytosis. Routine prophylactic phlebotomies in cyanotic patients pose the potential hazard of iron deficiency, cerebrovascular events, and decreased exercise tolerance [36, 65, 66]. In fact, venesections are hardly ever necessary today [67]. Hyperviscosity symptoms in cyanotic patients are often caused by iron deficiency or dehydration. Hyperviscosity symptoms include headache, light‐headedness, dizziness, blurred or double vision, paresthesias, altered cognitive functions, fatigue, tinnitus, and restlessness. Venesection should not exceed 1 unit of blood and should always be accompanied by volume replacement [64]. In fact, dehydration may exacerbate the hyperviscous state and aggressive volume replacement should be undertaken when hyperviscosity symptoms occur. The beneficial effects of phlebotomy are usually evident within 24 hours and reflect the increase in systemic blood flow induced by isovolumetric reduction in erythrocyte mass. Cyanotic ACHD patients are prone to iron deficiency because of increased iron requirements [36], recurrent phlebotomies, and blood loss (e.g., gastrointestinal, hemoptysis, menses) due to abnormal hemostasis (see later). Iron deficiency can increase blood viscosity through the production of microcytic hypochromic red blood cells, which are rigid and resistant to deformation. Moreover, iron deficiency may limit the physiologic rise in hemoglobin (relative anemia), resulting in impaired oxygen‐carrying capacity. Thus, annual screening for iron deficiency is important and is best performed by measuring serum ferritin and transferrin saturation [36]. Oral or intravenous iron can be used to treat iron deficiency, with close monitoring of hemoglobin levels to avoid excessive erythrocyte production. Cyanotic patients are at increased risk of both thrombotic events and bleeding. Hemostatic defects in cyanotic ACHD patients include thrombocytopenia, primary fibrinolysis, coagulation factor deficiencies, and disseminated intravascular coagulation. Bleeding in cyanotic patients can occur spontaneously (gingival bleeding, epistaxis, hemoptysis, or menorrhagia) or perioperatively/periprocedurally. Hemoptysis is especially common in patients with Eisenmenger syndrome and is the cause of death in 11–30% of patients [68]. Low–normal platelet counts and thrombocytopenia are common in cyanotic patients. The reduced platelet count can be caused by increased peripheral platelet consumption and decreased platelet life. It has also been suggested that megakaryocytes delivered into the systemic arterial circulation through right‐to‐left shunting cannot release platelets by cytoplasmic fragmentation, which would have normally occurred in the pulmonary circulation. Moreover, there are data suggesting that platelet function is abnormal (thrombasthenia) and that this improves with surgical repair of the underlying cardiac defect. Nevertheless, bleeding time in these patients is not increased, possibly due to the high blood viscosity and marked platelet activation. Disseminated intravascular coagulation has also been proposed as a mechanism responsible for the hemostatic abnormalities in cyanotic patients. The increased viscosity caused by the erythrocytosis may lead to a reduction in tissue perfusion and stasis. Widespread intravascular deposition of fibrin and platelets may lead to factor and platelet consumption and an increased risk of hemorrhage. Intravascular coagulation is probably nongeneralized, but localized to specific districts such as the pulmonary microcirculation. The reported increase in factor levels after heparin administration supports disseminated intravascular coagulation as an important mechanism for increased bleeding diathesis in cyanotic ACHD patients. Cyanotic CHD often results in congestive heart failure that may affect liver function and influence the production of coagulation factors. Even in the presence of normal liver function, gastrointestinal absorption of vitamin K could be impaired and affect production of factors II, VII, IX, and X. In the latter case, intramuscular administration of vitamin K could increase factor levels, whereas in the presence of abnormal liver function this will have little effect. Primary fibrinolysis has been reported in a few cyanotic patients, but its role is uncertain. Recently, evidence of increased sensitivity of cyanotic patients to activated protein C was reported, which could predispose patients to bleeding [69]. Other authors reported suppression of the thrombomodulin–protein C–protein S pathway that could predispose toward thrombosis in the microcirculation and subsequent disseminated intravascular coagulation phenomenon. Thrombosis in cyanotic heart disease is often a consequence of blood stasis occurring in dilated chambers, arrhythmias (atrial fibrillation or flutter), and prosthetic material (valves, pacemaker leads, conduits). Massive thrombosis of the pulmonary arteries was found in 29% of Eisenmenger patients in one study and thrombosis of the proximal pulmonary arteries was found in 21% of patients in another study [70]. Female sex and lower arterial saturations were associated with the risk of thrombosis in the pulmonary arteries. Pulmonary infarction appears to be the most common cause of hemoptysis in these patients [71]. The coexistence of hemoptysis and thrombosis makes clinical decision‐making on the management of anticoagulation arduous [68]. Treatment of the hemostatic dysfunction is not typically required. Moreover, administration of anticoagulants and antiplatelet agents in this group of patients remains controversial [72]. In our practice, indications for anticoagulation include atrial flutter or fibrillation, recurrent episodes of thromboembolic events in the absence of iron deficiency and dehydration, and the presence of mechanical heart valves or other high‐risk anatomy. Monitoring of anticoagulation in these patients should be meticulous [73]. Cyanotic ACHD patients requiring surgery are at high risk of perioperative bleeding due to the aforementioned hemostatic abnormalities and the presence of fragile mediastinal collateral arteries. Therefore, coagulation parameters should be accurately measured before surgery and fresh frozen plasma and platelets administered accordingly. Special attention should be made when assessing coagulation parameters in cyanotic patients with secondary erythrocytosis [71]. The increased hematocrit results in a decrease in plasma volume per unit of blood. Thus, the amount of anticoagulant in the vials used for collection of blood should be adjusted accordingly. Other measurements include administration of aminocaproic acid and tranexamic acid, meticulous hemostasis during sternotomy, application of fibrin glue to suture lines when necessary, and continuous ultrafiltration during cardiopulmonary bypass (CPB). Adult CHD patients are susceptible to a multitude of factors that may result in cumulative neurologic impairment. In the ACHD population, stroke may be related to paradoxical embolization through right‐to‐left shunts, previous palliative and corrective surgeries, infective endocarditis, cyanotic heart defects, or rheologic problems, as well as acquired risk factors for stroke [74]. Although there are numerous stroke case studies, comprehensive data on stroke in patients with ACHD are lacking. In adult patients with cyanotic heart disease, the risk of cerebrovascular thrombotic events was estimated at 14% (1/100 patient‐years) in one study, whereas in another study no cerebrovascular events occurred (112 patients, 748 patient‐years) [75]. In patients with ACHD who were <55 years of age, ischemic stroke was found to be approximately 9–12 times higher, and in patients who were 55–64 years of age the incidence of ischemic stroke was 2–4 times higher than in the general population. With regard to lesion categories, patients with severe and left‐sided lesions had the highest cumulative risk of stroke during adulthood. Heart failure, diabetes mellitus, and recent myocardial infarction were the comorbidities with the strongest predictive ability for stroke. Daliento and colleagues reported that 8% of Eisenmenger patients had a major cerebrovascular event by age 31 ± 16 years [76]. Systemic arterial hypertension, atrial fibrillation, and microcytosis are independent predictors of cerebrovascular events in cyanotic heart disease [65]. Heart failure, diabetes mellitus, and recent myocardial infarction were the strongest predictors of ischemic stroke. Multiple ischemic changes were identified by MRI in cyanotic patients with heart disease even in the absence of clinical history of major cerebrovascular events, atrial fibrillation, brain abscess, or prosthetic valves. Patients with a hematocrit exceeding 60%, oxygen saturation <85%, and decreased protein C activity were at higher risk of subclinical ischemic cerebral changes on MRI. However, Perloff and colleagues found no relation between stroke due to arterial thrombosis and hyperviscosity [77]. In fact, blood viscosity may have less of an effect on flow rates in the adult cerebral microcirculation, and intrinsic autoregulation may preserve blood flow in the face of hyperviscosity [78]. Additional research is required to see whether early detection of risk factors and advances in the management of ACHD may reduce this substantial stroke rate. The kidney is at high risk of long‐term negative impact, given the pathophysiologic changes that occur in the context of CHD, surgical intervention, CPB, postoperative critical care, and recurrent exposure to potential renal insults. Although there are a significant number of gaps in the knowledge related to the renal outcomes of children with CHD and children who have had cardiac surgery, current evidence demonstrates chronic kidney disease as an increasingly prevalent and important problem in these patients. Children with CHD have several risk factors for the potential development of chronic kidney disease (CKD) later in life, including pathophysiologic changes related to a structurally abnormal heart and circulation. These may include secondary erythrocytosis, cyanosis, chronic hypoxia, changes in renal blood flow and intraglomerular hemodynamics, and derangements in neurohormonal activation. Chronic cyanotic heart disease is associated with decreased glomerular filtration rate and proteinuria. This is secondary to hypercellular congested glomeruli with segmental sclerosis and basement membrane thickening [79]. In a recent study, 72% of patients with Eisenmenger syndrome were found to have a moderate or severe reduction in glomerular filtration rate [80]. Moreover, renal dysfunction is an established marker of adverse outcome in acquired and congenital heart disease (Figure 47.3) [80]. Care should be taken when performing cardiac catheterization with angiography in cyanotic patients who are at increased risk for contrast‐induced nephropathy. Patients requiring cardiac catheterization should be well hydrated prior to the procedure and a minimal amount of contrast agent should be used for imaging. Postcatheterization monitoring of urinary output is required and rehydration should be used judiciously. Patients with CHD should be recognized as a population at risk of developing CKD. The pathophysiology in CHD can lead to changes in the structure and function of the kidney. In addition, the presence of CHD concomitantly places patients at risk of exposure to factors that cause acute kidney injury, including CPB and nephrotoxins. Although limited, the current epidemiologic evidence suggests that renal dysfunction and CKD occur in patients with CHD with higher frequency than in the general population and are detectable early in follow‐up (i.e., during childhood). Despite their relatively young age, the best evidence suggests that approximately 30–50% of adult patients with CHD have significantly impaired renal function. The risk of CKD is higher with cyanotic CHD, but it is also present with noncyanotic CHD. There are currently no clear guidelines for clinicians in terms of renal assessment in the long‐term follow‐up after cardiac surgery in childhood. However, this population represents one in whom long‐term primary and secondary prevention strategies to reduce CKD occurrence and CKD progression could be instituted to significantly change outcomes. The sum of the data suggests that patients with CHD should be followed from an early age for the development of CKD. By instituting universally accepted interventions including stringent blood pressure control and treatment of proteinuria, there is an opportunity to mitigate CKD progression and negative renal outcomes. Figure 47.3 Unadjusted (Kaplan–Meier) cumulative mortality curves according to glomerular filtration rate (GFR) group. Patients with moderate or severe GFR reduction (<60 mL/min/1.73 m2) had a fivefold increased unadjusted mortality risk, which was apparent even from the first year of follow‐up. Source: Dimopoulos et al. 2008 / With permission of Wolters Kluwer Health, Inc. Acute gout occurs in 2% of adults with cyanotic heart disease. High uric acid levels require no treatment if gout is absent; acute symptomatic gout may be treated with colchicine. However, colchicine may produce vomiting or diarrhea, which requires discontinuation to prevent dehydration. Nonsteroidal anti‐inflammatory agents may be used with caution, acknowledging the increased risk of bleeding with their use. Pulmonary infarction due to pulmonary embolus may result in hemoptysis. Hemoptysis may also be caused by rupture of an enlarged friable bronchial artery. Prompt bronchoscopy should be performed to clear the airway and identify the site of the lesion. Measures to protect the contralateral bronchial tree are undertaken. CT or MRI may be beneficial in identifying pulmonary infarction and intraparenchymal hemorrhage. If bleeding persists despite bed rest and correction of coagulopathies, angiography to localize the site of bleeding is indicated. At the time of angiography, embolization of the vessel should be performed. If bleeding cannot be controlled with interventional catheterization techniques, then emergent pulmonary resection is required. As female patients with CHD reach reproductive age, the issue of pregnancy and labor naturally arises. Preconceptual counseling should start in adolescence, especially for high‐risk patients [81, 82]. Ideally, age‐appropriate counseling should be initially offered at or soon after sexual maturity in the patient’s teen years. Patients should be educated about the risks and the precautions to be taken, allowing them to make informed decisions regarding family planning. Counseling should include a discussion of the anticipated impact of pregnancy on maternal heart disease and the importance of pregnancy planning, including effective contraception options. The risk of maternal death is less than 1% for most patients with CHD. Certain conditions, however, are associated with significantly higher risk [81]. The modified World Health Organization (WHO) classification categorized patients into four pregnancy risk classes (classes I–IV) as determined by their medical condition. Patients in class I have no detectable increased risk of maternal mortality and either no increase or a mild increase in morbidity. Women in class II might have a small increase in maternal mortality or a moderate increase in morbidity with pregnancy, and those in class III might have a significant increase in maternal mortality or severe morbidity. Those in class IV, however, may carry an extremely high risk of maternal mortality or severe morbidity such that pregnancy is ill advised. These patients should be counseled to avoid pregnancy. If pregnancy is confirmed in a woman in WHO class IV, termination is advised and should be discussed. More recently, a prospective validation study reported that the modified WHO classification of maternal cardiovascular risk was the most reliable predictor of maternal cardiovascular complications, the most common of which are arrhythmias and heart failure. The recommendation to each patient, however, should be individualized. If a reparable lesion or clinical problem is identified at the time of prepregnancy counseling, a recommendation for directed therapies to address the problem is made. Maternal risk in pregnancy is dependent not only on the complexity of the primary cardiac lesion, but also on the presence of residual lesions and clinical sequelae such as heart failure, arrhythmias, or cerebrovascular events that contribute to overall risk. Several risk stratification scores have been developed to predict maternal risk during pregnancy. These risk scores have significant limitations, however, because they are highly population dependent. An important step in the management of ACHD patients who wish to become pregnant is prepregnancy assessment of cardiac status and counseling on the risks of pregnancy, intervention required before becoming pregnant, and the need for close follow‐up during and after pregnancy and labor. For the woman contemplating an immediate pregnancy, a more focused conversation is required about pregnancy risk and the possible need to undergo additional diagnostic cardiovascular evaluation to determine maternal risk, optimize maternal status, and treat or repair any residual defects or other problems before conception. The discussion should also include the possibility that pregnancy may contribute to a decline in maternal cardiac status that may not recover to baseline after pregnancy. A brief overview of the anticipated delivery plan (vaginal versus cesarean delivery, anesthesia) should also be discussed. A preconception visit with a maternal–fetal medicine obstetrician can be helpful, particularly for women at higher risk of complications. Genetic counseling may be particularly valuable to women for whom there is a significant risk of recurrence in offspring. The risk for recurrence of CHD varies widely from 3% to 50%, depending on the type of maternal heart disease [83, 84]. Inevitably, some women, regardless of the risks to their health, will decide to become pregnant. Ideally, the cardiovascular evaluation should occur before conception to minimize risks and optimize cardiac function. This process may include catheter or surgical intervention before gestation, surgical or medical treatment (including arrhythmias), and appropriate management of medication, including anticoagulation and angiotensin‐converting enzyme (ACE) inhibitors, which may harm the fetus. Patients with moderate to severe left‐sided obstructive lesions should be recognized and considered for balloon valvotomy or surgery before pregnancy. The choice of bioprosthetic or mechanical valve requires careful discussion with the patient. In general, metallic prostheses should be avoided in women of reproductive age, as anticoagulation poses a significant risk to the mother and/or the fetus. Some early reports suggested accelerated degeneration of bioprostheses during pregnancy [85–92]; however, this has not been verified by subsequent large series [93]. Nevertheless, it has been suggested that the appearance of accelerated degeneration associated with pregnancy might reflect the well‐established deterioration of bioprostheses in younger patients that has been reported in the medical literature. Mechanical prostheses are more problematic than tissue valves during gestation due to the increased risk of clotting [94, 95]. Careful pregestation assessment of aortic root size is important in women with Marfan syndrome, bicuspid aortic valve, and aortic coarctation associated with hypertension. Elective aortic root replacement should be considered in patients with large or enlarging aortic roots [96]. Similarly, women with repaired TOF, pulmonary regurgitation, and right ventricular enlargement may be considered for pulmonary valve replacement before pregnancy. In fact, recent studies have demonstrated an increase in right ventricular size with pregnancy, which persists after labor [96]. For the patient who presents for the first time with an unplanned pregnancy, the diagnostic workup must be individualized with consideration of the potential risk to both mother and fetus. The relative safety of any medication during pregnancy involves the comparative determination of maternal benefit versus potential fetal risk. It is important to provide genetic counseling to women with CHD on the potential for recurrence risk in the offspring, ideally before conception. Maternal CHD is a major determinant of risk to the fetus and the neonate, resulting in higher frequency of spontaneous abortions (ranging between 15% and 25%); higher frequency of recurrence of CHD (underscoring the need to offer fetal echocardiography to all pregnant women with CHD at 18–22 weeks); higher preterm birth rate (10–12%) in those with complex CHD; higher frequency of neonatal events such as small for gestational age, respiratory distress syndrome, interventricular hemorrhage, and neonatal death (27.8%); and higher perinatal mortality that may be more than fourfold higher than in the general population (<1%). Arrhythmia is a frequent complication of pregnancy in women with CHD and may cause significant hemodynamic compromise to both the mother and the fetus [97]. Many antiarrhythmic drugs cross the placenta and may adversely affect fetal development; the risk–benefit ratio must be considered in every case. In general, drugs are avoided in the first trimester unless necessary, but if they are required for arrhythmia control, several options are available. Additionally, direct current cardioversion is safe and may be used to treat hemodynamically unstable arrhythmias. Radiofrequency catheter ablation therapy, however, is discouraged and should be considered only in patients with drug‐refractory, poorly tolerated arrhythmias. Direct current cardioversion is safe during pregnancy, whereas antiarrhythmic drug therapy may have adverse effects and should be used with great care [98, 99]. Maternal risks include valve thrombosis, thromboembolic complications, heart failure, arrhythmias, endocarditis, and hemorrhage. Fetal and neonatal risks include fetal loss, still birth, preterm birth, growth restriction, and prematurity. The rates of complications vary in relation to the woman’s underlying cardiac condition, the function and location of the mechanical valve, and the type of anticoagulation used during pregnancy. Because of the high‐risk nature of these pregnancies, all women should be seen for preconception counseling by a cardiologist with expertise in pregnancy and valve disease. The management of anticoagulation is often problematic, especially in women with prosthetic heart valves. Anticoagulation options include vitamin K antagonists (e.g., warfarin), low molecular weight heparin, and unfractionated heparin. New direct oral anticoagulants are not used in patients with mechanical valves. Warfarin is a more effective anticoagulant in pregnancy, but it is also associated with increased fetal loss and embryopathy, especially when used between weeks 6 and 12 of pregnancy. Furthermore, there is a risk of fetal cerebral bleeding. Our current practice consists of low molecular weight heparin throughout the pregnancy combined with aspirin. We closely monitor levels by frequent assessment of anti‐Xa levels. The use of aspirin is safe until the week 35 of pregnancy in women with increased risk of thromboembolism, such as patients with a Fontan circulation, and in those at risk of paradoxical embolization across an intracardiac defect. The timing of pregnancy is particularly important for women with a systemic RV or a univentricular heart, as they are more likely to tolerate pregnancy earlier rather than later in their lives. In fact, progressive ventricular dysfunction in these patients is common and affects the risk and outcome of pregnancy. Despite all efforts for optimal medical management of pregnancy in patients with CHD, the need for surgical or catheter intervention during pregnancy may still arise. Most interventions involve women with an undiagnosed cardiac lesion, those lost to follow‐up, or those with an acute, life‐threatening situation. The most common indication for cardiac intervention during pregnancy is valvar heart disease, in particular mitral valve stenosis, which is usually secondary to rheumatic heart disease [100]. Other indications include severe aortic valve stenosis, critical pulmonary valve stenosis, acute dissection of the aorta, pacemaker insertion, and insertion of an inferior caval vein filter. CPB is associated with a high risk of fetal loss and should be only considered in women with symptoms refractory to medical treatment, for whom no percutaneous options are available. Resternotomy in patients who have undergone prior palliative or corrective procedures carries an increased risk. Lying just beneath or adherent to the posterior sternal table may be a dilated thin‐walled right atrium, an enlarged RV, a RV to pulmonary artery conduit, the aorta (in patients with transposition of the great arteries), or a markedly dilated aorta (common, for example, in TOF). Meticulous review of the previous operative notes, posteroanterior and lateral chest x‐ray, CMR imaging, and cardiac catheterization can aid in planning the resternotomy. The groin should be prepped and draped into the operative field should rapid access be required during resternotomy. The femoral vessels can be dissected before the resternotomy for rapid cannulation and institution of CPB if risk of injury to the right‐sided heart chambers appears high. Alternatively, femoral cannulation with institution of CPB may be accomplished before resternotomy to allow decompression of the heart, which may reduce the risk of injury. Peripheral cardiopulmonary artery bypass can be converted to central atrial–aortic bypass once the heart has been dissected free from the surrounding structures. This provides more effective venous drainage and reduces the risk of compartment syndrome due to reduced arterial flow in the limb. Some authors prefer to develop a dissection plane just behind the posterior sternal table under direct visualization before sternotomy is attempted. Once the sternum has been successfully opened, early identification of the aorta and atrium should be achieved so that rapid institution of CPB may be accomplished in the event of a misstep during dissection of the heart. During the course of the operation, the dissection plane should remain medial to the pericardium to avoid injury to the phrenic nerve. In many cases the pericardium has previously been harvested for intracardiac repair; therefore, the phrenic nerves are at increased risk for injury. Entry into the cardiac chambers is to be avoided to reduce the risk of paradoxical air embolus occurring through residual intracardiac shunts. If entry into the cardiac chambers occurs, suture closure of the defect should be performed. Also during dissection, attention should be paid to the patient’s hemodynamics. Cardiac output is reliant on adequate volume loading of the heart. Most patients are volume depleted secondary to their nothing by mouth status. In addition, retraction on the heart during dissection may result in severe hemodynamic instability. If exposure for dissection is difficult, then institution of CPB should be considered. With careful preoperative planning and great care during resternotomy, poor outcomes can be avoided. Chronic cyanosis may lead to the development of multiple thin‐walled mediastinal collateral vessels. These vessels may become problematic at the time of sternotomy and during the operation. They do not coagulate well and may need to be sutured to avoid unnecessary persistent blood loss during preparation of the heart for CPB. During the operation, systemic to pulmonary collaterals may produce excessive pulmonary venous return, making visualization of intracardiac anatomy difficult and resulting in myocardial distention and wash‐out of cardioplegic solution and myocardial rewarming. In these circumstances a left atrial vent will be required. Occasionally, systemic cooling and periods of low‐flow CPB will be required. Large collateral vessels identified on preoperative cardiac catheterization should be occluded at the time of cardiac catheterization (transcatheter coil occlusion). If cardiac catheterization is not performed or coil occlusion is unsuccessful, suture ligation of the collaterals should be performed during the initial stages of the operation to avoid complications during the procedure. The classic Blalock–Taussig–Thomas (BTT) shunt involves ligation of the subclavian artery with anastomosis of the subclavian to the pulmonary artery. The radial pulse is absent on the side of the shunt; therefore, arterial monitoring must be performed on the contralateral side. The modified BTT shunt is an interposition polytetrafluoroethylene (PTFE) graft between the subclavian or innominate artery and the pulmonary artery. Arterial blood pressure monitoring should be performed on the opposite side of the shunt. Blood flow through the shunt, and thus the pulmonary blood flow, is dependent on systemic blood pressure. During the induction of anesthesia, care must be taken to prevent systemic hypotension, which can result in both systemic and pulmonary hypoperfusion. Aspirin is often prescribed in patients with BTT shunts and should be discontinued 5 days before reoperation. The shunt is dissected free from the surrounding tissue before institution of CPB. Injury to the shunt during the dissection may have disastrous consequences if the shunt is the only source of pulmonary blood flow. At the start of CPB, the shunt is ligated and divided to avoid excessive pulmonary blood flow and prevent systemic hypotension during CPB. Pulmonary artery distortion or stenosis may occur at the site of the PTFE–pulmonary artery anastomosis. These anatomic abnormalities are repaired using arterioplasty techniques to restore unobstructed blood flow to the lungs. The classic Glenn shunt is an end‐to‐end anastomosis between the divided right pulmonary artery and the superior caval vein. Therefore, all superior caval vein blood flow is directed to the right lung. The bidirectional Glenn is an end‐to‐side anastomosis of the superior caval vein to the superior margin of the right pulmonary artery, thereby maintaining continuity between the right and left main pulmonary arteries. This permits bidirectional flow from the superior caval vein to the right and left lungs. With both the classic Glenn and the bidirectional Glenn shunt, the inferior caval vein is left intact, resulting in right‐to‐left shunting and hypoxemia. As previously discussed, chronic hypoxemia leads to erythrocytosis, abnormalities of hemostasis, development of collateral circulation, and systemic to pulmonary arterial shunts. If patients with classic Glenn shunts become candidates for the Fontan operation, the classic Glenn shunt is taken down, pulmonary artery continuity is reestablished, and a bidirectional Glenn is constructed. During dissection of the Glenn shunt, care must be taken to avoid injury to the phrenic nerve. Pulmonary artery banding protects the pulmonary circulation from excessive blood flow during infancy, thereby reducing the risk of pulmonary arterial hypertension. A pulmonary artery band results in focal supravalvar stenosis of the main pulmonary artery. At the time of reoperation, repair of the stenosis must be addressed. Repair is typically performed by pericardial or prosthetic patch arterioplasty. If the band has migrated distally on the artery, then bilateral pulmonary artery stenoses or distortion of the pulmonary arteries may occur. The anatomic defect can be repaired with pericardium, homograft, or prosthetic material. Planning for an anesthetic procedure requires in‐depth knowledge of the cardiac anatomy and physiology and the patient’s medical history. Successful delivery of anesthesia requires modification of techniques to account for specific pathophysiologic states. Adequate premedication is needed to prevent anxiety, tachycardia, and increased myocardial demands. In patients with physiology dependent on preload (Fontan patients), avoidance of myocardial depression, vasodilatation, and hypotension is critical for maintenance of hemodynamic stability. Moreover, large tidal volumes or high levels of positive end‐expiratory pressure should be avoided to prevent a reduction in pulmonary blood flow. Hypoxemia and acidosis may also induce pulmonary vascular constriction and thus reduce pulmonary blood flow. A balance between pulmonary and systemic blood flow may be obtained by adjusting the fraction of inspired oxygen and ventilation. Patients with increased pulmonary blood flow should be maintained on minimal oxygen, with permissive hypercapnia and mild acidosis to prevent excessive pulmonary blood flow producing dilatation and failure of the systemic ventricle. The degree of invasive monitoring required during anesthesia depends on the severity of the congenital cardiac defect and associated physiology. Continuous electrocardiographic monitoring is essential for identifying arrhythmic or ischemic events, especially during the onset of general anesthesia and before the institution of CPB. Pulse oximetry and end‐tidal CO2 monitoring assess the adequacy of oxygenation and ventilation throughout the procedure. Particular attention to the balance between oxygenation and ventilation is important in those patients in whom the pulmonary flow, filling of the systemic ventricle, and cardiac output are dependent on the resistance of the pulmonary vascular bed (i.e., Glenn, Fontan). Systemic arterial catheters provide continuous blood pressure monitoring and arterial access for blood gas analysis throughout the course of the operation. Placement of the catheter may be problematic due to numerous prior cannulations, arterial cut‐downs, and the presence of systemic to pulmonary shunts (i.e., BTT shunt). Use of the femoral or lower‐extremity arteries may be limited due to associated coarctation of the aorta. Central venous pressure monitoring is important for assessing preload and the fluid management in patients who require high central pressures to maintain adequate pulmonary perfusion. Insertion of central venous catheters in patients with ACHD may be difficult due to abnormal venous anatomy and thrombosis of previously cannulated central veins. Extreme care should be taken to avoid systemic air embolism during insertion of central lines in patients with right‐to‐left shunts. Pulmonary artery catheters are not used routinely, but may be beneficial in patients with anticipated postoperative hemodynamic instability. Patients with left‐to‐right shunts may benefit from a mild increase in PVR induced through a low fraction of inspired oxygen (lowest acceptable level to maintain sufficient partial pressure of arterial oxygen), and hypercapnia with mild acidosis. This limits excessive pulmonary blood flow and distention of the systemic ventricle during anesthesia. In patients with right‐to‐left shunts due to a large intracardiac communication and fixed right ventricular outflow tract (RVOT) obstruction, which protects the pulmonary vascular bed from excessive blood flow, pulmonary blood flow can be improved by increasing systemic vascular resistance. In patients with pulmonary arterial hypertension resulting from an intracardiac shunt, the maintenance of a balance between systemic and PVR during surgery is vital. Factors that may affect that balance include hypercarbia, hypoxia, atelectasis, uncontrolled vasodilation by anesthetics, altered intrathoracic pressures by artificial ventilation, and hypovolemia. A combination of meticulous fluid administration, norepinephrine infusion, and inhaled nitric oxide can preserve a stable circulation. High catecholamine levels result in increased dynamic RVOT obstruction, leading to worsening cyanosis in patients with TOF. Adequate preoperative premedication is required to limit the catecholamine surge associated with anxiety and pain. Use of prebypass inotropic agents may also increase the dynamic right ventricular outflow obstruction and result in hemodynamic deterioration. Hypovolemia and vasodilating agents can also worsen right‐to‐left shunting. Beta‐adrenergic antagonists decrease dynamic right ventricular obstruction and can reduce the risk of “tet” spells during the perioperative time period. In severe cases of acute cyanosis, phenylephrine may be used to increase systemic vascular resistance, reducing right‐to‐left shunting, which may lead to hemodynamic improvement. If supportive and pharmacologic measures fail, rapid institution of CPB is required. On completion of the operation, the patient is transported to the intensive care unit (ICU). It is during this time of decreased monitoring that complications may occur. Portable monitors are used during transport to monitor blood pressure, oxygen saturation, and the ECG. All intravenous infusions should be maintained during transport. Equipment for reintubation and medication for the treatment of cardiac arrest should be immediately available. On arrival at the ICU, mechanical ventilation is reinstituted, temporary monitoring is converted to permanent monitoring, and infusion of medication is established and confirmed. Adequate handover is also fundamental for ensuring a smooth transition. Blood is drawn for routine laboratory tests (e.g., complete blood count, electrolytes, coagulation factors, arterial blood gases). Electrolyte abnormalities and coagulation deficiencies should be corrected promptly. Portable chest x‐ray is obtained to confirm proper position of the endotracheal tube and rule out hemothorax or pneumothorax. Adequate warming begins with warm blankets, forced warm air blankets, or radiant heat lamps, as body heat is often lost during transport from the operating room to the ICU. The respiratory management of ACHD patients may differ significantly from that of adults undergoing repair of acquired cardiac lesions. In patients with ACHD, alterations in respiratory mechanics can have profound effects on hemodynamics. An increase in intrathoracic pressure can affect systemic venous return, right ventricular ejection, left atrial filling, and cardiac output. Postoperative pulmonary arterial vasodilatation is desirable in patients with previous right‐to‐left shunts and pulmonary arterial hypertension. The goal of ventilator management is to maintain partial pressure of arterial oxygen between 100 and 150 torr, mild respiratory alkalosis with a pH of 7.50–7.60, and a CO2 between 30 and 35 torr. Sedation and analgesia are administered using intermittent or continuous infusions of morphine and midazolam to help prevent transient pulmonary hypertensive crises associated with painful stimulation. In cases of severe pulmonary hypertension, temporary paralysis with pancuronium bromide may be beneficial. Ventilator strategies differ for patients with shunt‐dependent pulmonary blood flow. These patients require a reduction in oxygen saturation (75–80%) and an increase in CO2 (45–50 torr). This management protocol improves systemic blood flow by increasing PVR, thereby reducing pulmonary blood flow and increasing systemic flow [101]. In patients with a bidirectional Glenn, hypoventilation (PCO2 48–52 torr) has been shown to improve pulmonary blood flow [102]. Hypoventilation results in cerebral vascular vasodilatation, thus increasing cerebral blood flow and the volume of blood return to the pulmonary arteries through the superior caval vein. Fontan physiology is dependent on passive pulmonary blood flow. Low tidal volumes, minimal positive end‐expiratory pressure, and early extubation minimize respiratory inhibition of passive pulmonary blood flow [103]. Close cardiovascular monitoring is required during the initial postoperative period. Hemodynamic changes occur frequently due to rewarming, vasodilatation, variations in acid–base status, and changes in the catecholamine levels in response to the level of sedation and pain control. Continuous monitoring of arterial blood pressure, central venous pressure, cardiac output, oxygen consumption, and body temperature is extremely helpful. Hypotension often occurs during rewarming. Hypovolemia may occur due to fluid shifts from the intravascular space to the extravascular space. Postoperative hypothermia may also cause intravascular volume depletion, but can be associated with normal systemic blood pressure due to peripheral vasoconstriction. Crystalloid, colloid, or blood may be used for fluid replacement. Mild sodium and water retention occurs with CPB, resulting in increased body weight. Spontaneous mobilization of interstitial fluids and diuresis typically occur once the patient is rewarmed and capillary leak has subsided. Diuretics may be used to enhance the diuretic phase, depending on the patient’s clinical status. Hypokalemia and hypomagnesemia often occur due to hemodilution. Potassium should be replaced through central lines to maintain serum levels above 3.5 mEq/L. Magnesium should be normalized to reduce the incidence of atrial and ventricular arrhythmias [104]. Metabolic acidosis should also be promptly corrected, as it may result in decreased cardiac function, impaired tissue perfusion, and reduced efficacy of inotropic agents. Causes of metabolic acidosis (e.g., hypothermia, hypovolemia, ischemia) should be addressed. Treatment with sodium bicarbonate (1 mEq/kg) may be required while correcting the cause of the acidosis. There is no single sign or symptom diagnostic of low cardiac output syndrome. Frequent postoperative assessments and a low threshold of suspicion are imperative. Assessment includes capillary refill time, absent or weak peripheral pulses, cool mottled skin, decreased urinary output, acid–base balance, and core body temperature. Treatment of low cardiac output is based on identification and reversal of the etiology. Cardiac output is the product of heart rate and stroke volume. Stroke volume is the difference between end‐diastolic volume and end‐systolic volume. Diastolic volume is dependent on preload and compliance of the ventricle. Systolic ejection is a function of afterload and contractility. Fluctuations in heart rate, preload, afterload, and contractility often occur during the postoperative period. The interrelationship between the determinants of cardiac output can be manipulated to maintain adequate tissue perfusion. In general, low cardiac output may be caused by (i) alterations in heart rate and rhythm; (ii) decreased preload due to hypovolemia, cardiac tamponade, or excessive intrathoracic pressures; (iii) increased afterload from pulmonary arterial hypertension and increased systemic vascular resistance; (iv) decreased contractility from acidosis, myocardial injury/ischemia due to poor myocardial protection during the operation, ventriculotomy, or acidosis; and (v) pump failure with myocardial injury. Mechanical assist devices were initially developed for the treatment of postcardiotomy cardiogenic shock. In recent years, however, they have also been used to provide temporary cardiac support in patients with irreversible cardiomyopathy until a donor heart becomes available for transplantation (i.e., “bridge to transplantation”) [9, 105, 106]. Less commonly these devices may be used in patients with reversible heart failure as a “bridge to recovery” by unloading the ventricle while it is in the recovery phase following an acute insult such as viral cardiomyopathy. Destination therapy refers to the use of some of these pumps to permanently support the heart in patients who are not transplant candidates because of major contraindications. Several types of devices currently exist, and major technologic advances in their development have been made in recent years. The basic left ventricular assist device (LVAD) configuration consists of an inflow or drainage cannula placed into the left atrium (via the left atrial appendage or right superior pulmonary vein/left atrial wall junction) or the left ventricle through the apex. The pump, which may lie in the paracorporeal or intracorporeal position, is filled by the inflow cannula and then pumps the blood in either a pulsatile or nonpulsatile manner into the outflow cannula, which is connected directly to the ascending aorta. In cases where the patient has established severe biventricular failure, a biventricular assist device (BiVAD) is used with the inflow cannula of the right‐sided (RVAD) device draining the right atrium and the outflow cannula anastomosed to the main pulmonary artery. Similarly, a BiVAD may be required when right ventricular dysfunction or failure becomes unmasked shortly after the start of LVAD support. Once LVAD or BiVAD support has been established and the hemodynamics have been stabilized, the patient is weaned off catecholamine‐based inotropes to minimize the oxygen consumption and metabolic stress imposed on the unloaded ventricle, thus facilitating the process of recovery. Further assessment to determine the likelihood of recovery is made with regular multidisciplinary discussions between the cardiac surgeon, cardiologist, and intensivist. Close monitoring of the central hemodynamics (Swan–Ganz catheter), mixed venous oxygen saturations, end‐organ perfusion (renal output), metabolic status, and transesophageal echocardiogram parameters should all be used to determine whether the ventricle is showing convincing signs of recovery. The level of anticoagulation should be intensified during a 6–8‐hour period of “low flows” (<1.2 L) as part of the trial of weaning protocol. Once the decision has been made to remove the device, inotropes are reestablished to support the patient in the early postexplant period. Short‐term mechanical circulatory assist devices are designed for a wide range of clinical conditions, ranging from prophylactic insertion for high‐risk invasive coronary artery procedures to the management of cardiogenic shock, acute decompensated heart failure, or cardiopulmonary arrest. These devices are often placed in the catheterization laboratory, but in some cases, such as an intraaortic balloon pump, can be placed in the ICU. Patients who develop postcardiotomy cardiogenic shock or acute decompensation of background chronic heart failure are often very ill and sometimes in extremis. These patients can be successfully rescued by the expeditious use of short‐term mechanical assist devices. Ideally a simple device is chosen that can be inserted relatively easily and often without the need for CPB support. This helps to minimize the inflammatory response and reduce postoperative bleeding. Furthermore, the management of the pump should be user‐friendly for the medical and nursing staff on the ICU. The Impella® device (Abiomed, Danvers, MA, USA) device has in the past been used successfully in patients requiring temporary support (a few days to a few weeks). It provides either univentricular or biventricular pulsatile mechanical support. The pump, which lies extracorporeally, consists of a 100 cc inflow and outflow polyurethane bladder separated by a trileaflet valve. The upper (atrial) chamber fills passively by gravity, and as the inflow bladder fills the console detects the displacement of air around the bladder and sends a bolus of compressed air to the lower (outflow) chamber, pumping blood back to the patient. As the upper atrial bladder fills passively by gravity, preload is controlled simply by raising and lowering the level of the Impella pump. Anticoagulation with systemic heparin is mandatory. The recognized complications include bleeding, respiratory failure, renal failure, neurologic deficit, and infection [107]. Several centers have reported good results with this device in the setting of postcardiotomy heart failure, with discharge rates of 50–59%, and for bridge to transplantation of 50% [108, 109]. More recently the CentriMag® (Levitronix, Waltham, MA, USA) ventricular assist device (VAD) has been used by many centers to provide temporary support for patients with acute decompensated heart failure. This device consists of a magnetically levitated rotating impeller without any mechanical bearings. This unique design minimizes trauma to the blood elements, even at high flows. Cannulation is relatively simple and can often be done without CPB. The cannulae are exteriorized and connected to the pump, which sits at the bedside on a retort stand next to the relatively small console. Potential flow rates of up to 9.9 L/min can be achieved at 5500 rpm. There are no flexing valves, diaphragms, or sacs, and the unique design helps to minimize the risk of blood stagnation, turbulence, and component failure. The degree of hemolysis associated with the CentriMag VAD, as measured by plasma‐free hemoglobin levels, has been shown to be significantly lower compared to other similar devices. The CentiMag VAD has been used successfully in the setting of bridge to transplantation and as a bridge to recovery for postcardiotomy cardiogenic shock [110, 111]. The Thoratec pneumatic Ventricular Assist Device (pVAD; Thoratec Laboratories, Pleasanton, CA, USA) is a pneumatically driven, pulsatile assist device that can be used to provide medium‐ to long‐term support. This VAD is indicated as both a bridge‐to‐transplantation and a bridge‐to‐recovery device. It can provide univentricular and/or biventricular support. The inflow and outflow ports contain Bjork–Shiley tilting disc valves to maintain unidirectional flow. The external position of the pump allows device exchange in cases of malfunction, thrombus, or infection, and use in smaller patients who are poor candidates for implantable devices. While on Thoratec VAD support, patients require systemic anticoagulation and can be ambulatory when connected to a mobile pneumatic console [112–114]. The Thoratec implantable VAD (IVAD) is a small, simple, and versatile IVAD with the same features as the Thoratec pVAD, except that longer support is anticipated due to the intracorporeal position. The HeartMate XVE® LVAD (Thoratec Laboratories) is a totally implantable, pneumatically or electrically driven dual‐chamber pump in titanium housing. The pump itself is positioned within the left upper quadrant of the abdomen or preperitoneally without entering the abdomen. The metallic side of the blood chamber is made of sintered titanium microspheres and this surface promotes the development of a neointima, which minimizes clot formation. Recipients of the device are therefore given only antiplatelet agents and do not require anticoagulation. This device can generate up to 8 L/min of flow and allows the patient to be mobile and indeed be sent home while awaiting a heart transplant. The HeartMate II® LVAD (Thoratec Laboratories) is a miniaturized rotary pump with axial flow design that represents a second generation of implantable assist devices. It is designed to be small, portable, and offers reliable long‐term outpatient left ventricular support. The system consists of an internal blood pump with a percutaneous lead connected to an external system, driver, and power source. The pump itself is implanted preperitoneally below the left costal margin and under the rectus abdominis muscle. The inflow cannula is placed into the left ventricular apex and tunneled through the diaphragm at the costophrenic angle into the preperitoneal pocket. The outflow cannula traverses across the midline under the sternum and is anastomosed to the ascending aorta. Miller and colleagues reported the results of a prospective multicenter study in 133 patients with end‐stage heart failure who were on the waiting list for heart transplantation and were bridged using the HeartMate II device [115]. The survival rate during support was 75% at 6 months and 68% at 12 months. At 3 months there was a significant improvement in functional status and in quality of life. Major adverse events included postoperative bleeding, stroke, right heart failure, and percutaneous lead infection. More recently, John and coworkers reported an improved 6‐month survival of 86.9% and a 3% incidence of device malfunction in a mean duration support of 6 months [116]. The US Food and Drug Administration (FDA) has already approved its use as bridge‐to‐transplant therapy. In addition, the HeartMate II has demonstrated great device reliability and significantly reduced device noise. It also has low thrombogenicity and low thromboembolic risk, which make it a good device option for destination therapy [117]. Currently, there is an ongoing trial in patients receiving LVADs as destination therapy comparing the use of HeartMate XVE and HeartMate II that will elucidate specific indications. The DeBakey LVAD (MicroMed Technology, Houston, TX, USA) is a small rotary pump with similar characteristics to the HeartMate II. Worldwide clinical experience has shown that this device is suitable for both bridge‐to‐transplantation and destination therapy. The success as a bridge to transplantation, reported in 150 patients, was between 50% and 66%, and device malfunction was observed in 3% of the population [118]. Multicenter, nonrandomized bridge‐to‐transplantation trials and a randomized device therapy trial (HeartMate XVE vs. DeBakey) are ongoing. The Jarvik 2000® (Jarvik Heart, New York, NY, USA) is a miniature axial flow pump (diameter 2.4 cm; length 5.5 cm). The pump is placed within the left ventricle (LV), different from the HeartMate II (placed in the abdomen in a preperitoneal position) or the DeBakey LVAD (placed within the thorax in the pericardial space). The avoidance of a pump pocket decreases the incidence of serious device‐related infections. The Jarvik 2000 is associated with less infections than the HeartMate LVAD. It also requires anticoagulation; however, the risk of hemolysis is minimal. Clinical experience has demonstrated that this device provides safe and effective therapy for patients with heart failure, both as bridge‐to‐transplantation and destination therapy [105, 119]. This pump optimally performs when it acts as a true assist device by partially unloading the LV, thereby allowing reverse remodeling and native ventricular ejection to contribute to the remainder of the cardiac output. The first patient to receive the Jarvik 2000 as destination therapy was supported continuously for more than 6 years [120]. No internal device components failed during an accumulative support time of 59 years. Furthermore, the freedom from LVAD system failure due to external components was 95% at 1–4 years [121]. Clearly, such proven reliability and durability make the Jarvik 2000 promising for long‐term use in selected patients. ASDs are the third most common form of ACHD. There are several types of ASD, according to the region of the atrial septum involved. Secundum ASDs are the most common and can be amenable to percutaneous closure, provided that their anatomy is suitable. Primum, sinus venosus, and coronary sinus ASDs all require surgical closure. Common presenting symptoms in the adult are arrhythmia and exercise intolerance with dyspnea on exertion, while many are identified during routine medical screening. Repair of an ASD is usually indicated when significant left‐to‐right shunting results in right ventricular dilation associated with volume overload. Other indications for closure include documented paradoxical embolism and platypnea–orthodeoxia syndrome [122]. In patients with unrepaired ASD, the incidence of atrial arrhythmias increases with age and has been reported to be as high as 83% in patients who are more than 50 years of age [123]. Atrial arrhythmias may precipitate atrial thrombosis and the potential for paradoxical emboli. Moreover, as patients become older, the incidence of congestive heart failure due to right heart failure increases. Tricuspid regurgitation has been identified by echocardiogram in 28% of patients who are more than 50 years of age and in 50% of patients more than 60 years of age [123]. The real breakthrough with transcatheter ASD closure came with the development of the Amplatzer® Septal Occluder (AGA Medical Corporation, Plymouth, MN, USA) in the mid‐1990s. This device consists of a nitinol wire mesh that is tightly woven into two disks with a connecting waist between them that corresponds to the approximate thickness of the interatrial septum. Although many other devices have been reported, the other mainstream device in clinical use today is the HELEX® septal occluder (W.L. Gore, Flagstaff, AZ, USA). This device consists of a single nitinol wire covered by an ultrathin membrane of expanded PTFE. In its occlusive configuration, the device forms two round flexible discs that straddle the septum. The first clinical reports with the Amplatzer Septal Occluder were published in the late 1990s and early 2000s [124–126]. In 2007 Patel and associates studied 113 patients who were more than 40 years of age and demonstrated that ASD closure using the Amplatzer Septal Occluder in these patients was safe and effective with minimal complications [127]. There was a remarkable decrease in symptoms, which included fatigue, dyspnea, exercise intolerance, and palpitations, as well as a decrease in the size of the RV. In another study, percutaneous ASD closure was found to result in early and remarkable cardiac geometric improvements that nearly reverted the right‐to‐left volumetric imbalance. Most of this remodeling occurs within a few weeks after ASD closure. Transcatheter ASD closure is also safe and effective in patients who are more than 60 years of age, and right ventricular remodeling has been reported in this age group as well [128–130]. Numerous subsequent reports have confirmed these data [131–134]. Patients who do not meet the criteria for transcatheter closure of the ASD require CPB for surgical closure. Anatomic indications for surgical closure usually include a stretched secundum ASD with a diameter of more than 36 mm, inadequate atrial septal rims, and proximity of the defect to the AV valves, the coronary sinus, or the caval veins [135]. The analysis of the American College of Cardiology IMPACT Registry® showed that a deficient retroaortic rim is not a contraindication for a percutaneous approach, as it had no effect on ASD device placement success or immediate outcome [136]. All patients who are more than 40 years of age undergoing surgical or transcatheter ASD closure should be also assessed for possible coexisting acquired heart disease, including coronary artery disease [137]. Primary suture closure, autologous pericardial patch closure, or prosthetic patch closure can be used. Minimally invasive direct access surgery also has been used for ASD closure. Byrne and colleagues reported 34 cases of minimally invasive ASD closure [138]. Access to the mediastinum was obtained through a right parasternal, submammary, or upper hemisternotomy incision. There were no operative deaths, but 12% of patients developed major complications (1 adult respiratory distress syndrome, 1 ASD recurrence, 1 upper extremity deep venous thrombosis, and 1 reoperation for bleeding). As experience in minimally invasive cardiac procedures increases, safety and efficacy have improved. Bichell and colleagues reported ASD closure through a 3.5–5.0 cm midline incision with division of the xiphoid process alone or by opening the lower portion of the sternum. No early deaths or major complications were reported, with a very brief hospital stay (mean 2.7 days) [139]. Standard open median sternotomy remains a safe and effective approach to the mediastinum for ASD closure. Following bicaval cannulation, the patient is cooled to 32 °C and the heart is arrested with cold‐blood antegrade cardioplegia. A right atrial atriotomy is then performed, taking care not to injure the crista terminalis, which may lead to an increased risk of postoperative atrial arrhythmias. For small defects or defects with redundant septum primum, the ASD is closed primarily by a two‐layer running suture technique. If the defect is too large, or adequate septum primum is not present for a tension‐free primary repair, pericardial or prosthetic patch closure is performed. Once de‐airing maneuvers have been accomplished, the patient is weaned from CPB. In patients with associated mitral valve disease, valve repair or replacement is performed through the ASD before closure. Surgical outcomes of ASD repair in adults are excellent. Perioperative mortality is less than 2% and morbidity (primarily atrial arrhythmias) is 8–13% [140–142]. Long‐term mortality is 1–2% and morbidity is 12–13% for a mean follow‐up of 9.6 and 7.5 years, respectively [123]. Improvement in NYHA functional class is reported in most patients. Patient age >40 years at the time of closure is considered an independent risk factor for the development of postoperative atrial flutter or fibrillation [142]. Postoperative embolic events have been reported in 4% of patients undergoing either primary or patch closure of ASD [123]. Long‐term follow‐up of adult patients following closure of ASD (mean 42.2 years, range 18.5–74.9 years) revealed that 22% of late deaths were due to stroke, occurring in patients with atrial flutter or fibrillation [143–146]. Stroke was also the main cause of death in patients who had undergone closure of ASD after the age of 60 years [147]. Because of the risk of postoperative emboli, anticoagulation should be maintained for three months following closure of ASD. Anticoagulation is continued in patients with persistent atrial arrhythmias. For patients with preoperative atrial flutter or fibrillation, the right‐sided maze or Cox maze III operation has been advocated in addition to ASD closure [148–150]. The management of adult patients with an ASD is determined by a number of factors, including symptoms, age, size of the defect, associated lesions, and PVR. Patients with a significant ASD with signs of right heart dilatation and a pulmonary artery systolic or mean pressure of less than 50% of the corresponding aortic pressures should be offered elective closure, irrespective of age. Young adults and older asymptomatic patients with indications for intervention should undergo closure of the ASD on diagnosis to prevent long‐term sequelae. If left untreated, the risk of death is 25% by 27 years of age, 50% at 36 years, 75% at 50 years, and 90% at 60 years [151]. Death occurs due to the sequelae of prolonged increased pulmonary blood flow, pulmonary arterial hypertension, atrial arrhythmias, embolic events, and congestive heart failure. The management of unrepaired ASDs in patients over the age of 60 years, especially when significant comorbidities exist, remains controversial. A few studies have reported percutaneous closure of ASD in elderly patients, with conflicting results [152–156]. Takaya and colleagues have recently assessed long‐term outcome after transcatheter ASD closure in patients who were >75 years of age. They showed that the outcome is similar to that in the other relatively younger age groups. There was an improvement in NYHA classification and right ventricular remodeling [157]. In fact, ASD closure can result in abrupt elevation of left ventricular overload, leading to left ventricular dysfunction and acute pulmonary edema, especially in patients with impaired systolic or diastolic left ventricular function [154, 155]. Several studies have reported successful transcatheter ASD closure in elderly patients who presented with normal left ventricular function, with favorable cardiac remodeling and improvement of functional class [128–130, 153, 156]. A recent study showed that echocardiographic estimation of left ventricular diastolic dysfunction did not predict the clinical course in elderly patients undergoing transcatheter ASD closure [156]. For the assessment of diastolic dysfunction, patients who were thought to be at high risk of developing hemodynamic compromise on ASD closure (moderate to severe elevated pulmonary pressure, impaired ventricular diastolic function, and modest left ventricular size) underwent transient balloon occlusion of the ASD with repeated measurements of pulmonary wedged pressure, left ventricular end‐diastolic pressure, systemic arterial pressure, and cardiac output. No cases of postoperative acute pulmonary edema were reported. Fenestrated ASD closure in patients with diastolic dysfunction is safe and may be better tolerated in selected patients than complete ASD closure [158]. Patients with an ASD and suspected pulmonary arterial hypertension should undergo right heart cardiac catheterization to measure pulmonary artery pressures. Pulmonary hypertension is a complication of the prolonged increase in pulmonary blood flow due to the ASD. Pulmonary artery hypertension and pulmonary artery resistance increase significantly between the age groups of 18–40 years and 40–60 years, with no further increase in patients >60 years [159]. The incidence of pulmonary arterial hypertension (mean pulmonary artery pressure >25 mmHg) is variable, ranging from 9% to 33% [159]. Gatzoulis and colleagues showed that the outcome of surgical repair was independent of pulmonary artery pressure in the presence of a left‐to‐right atrial shunt [140]. However, the patient with the highest pulmonary artery pressure in their study had a mean pressure of 45 mmHg. In contrast, the experience of Vogel and colleagues with patients who had repair of ASD and pulmonary arterial hypertension was not as encouraging. In their series there was one death (mean pulmonary artery pressure 68 mmHg, Qp : Qs 1.8 : 1) and two patients were listed for heart transplantation (mean pulmonary artery pressures 55 mmHg and 76 mmHg, respectively) [159]. Balint and colleagues performed a similar study with patients undergoing percutaneous ASD closure and moderate to severe pulmonary arterial hypertension, defined as moderate (50–59 mmHg) or severe (≥ 60 mmHg) according to the right ventricular systolic pressure (RVSP) [160]. Early follow‐up showed no deaths and an overall decrease in the RVSP from 57 (11) mmHg to 51 (17) mmHg (p=.003), whereas late follow‐up showed a decrease in the overall mean RVSP, but only 44% of patients had complete normalization of pressures (defined as RVSP <40 mmHg). In addition, two deaths occurred during late follow‐up, one due to bowel obstruction and the other due to recurrent pulmonary embolism. ASD closure in patients with pulmonary arterial hypertension remains a controversial issue. In the past operability in this group of patients was often decided based on lung biopsy. Currently, it relies on cardiac catheterization and the degree of vasoreactivity of the pulmonary circulation by means of inhaled 100% oxygen or nitric oxide, intravenous epoprostenol, or adenosine. In patients with evidence of vasoreactivity during acute testing but high PVR and bidirectional shunting, balloon test occlusion may provide additional information on the suitability for closure and the possible postprocedural outcome [39]. Detailed history, physical examination, resting oxygen saturations, chest x‐ray, ECG, and echocardiography are also important in the clinical decision [161]. In our practice, left‐to‐right shunting, that is to say in the absence of reversal of shunting and thus absence of resting or exercise‐induced cyanosis, is a favorable marker for operation. Sinus venosus ASD is a much rarer entity than secundum ASD. Commonly, the defect occurs at the junction between the superior caval vein and the right atrium. Typically, there is associated partial anomalous drainage of the right superior pulmonary vein into the lateral aspect of the superior caval vein at the level of the defect. When compared to patients with secundum‐type ASD, patients with sinus venosus ASD have a threefold greater incidence of pulmonary hypertension (26% vs. 9%) and a fourfold greater incidence of increased pulmonary artery resistance (16% vs. 4%) [159]. Pulmonary artery hypertension and increased pulmonary artery resistance occur at an earlier age in patients with sinus venosus‐type defects than in those with secundum defects. Therefore, adults with sinus venosus ASD should undergo right heart catheterization to rule out severe pulmonary arterial hypertension, which would preclude closure of the defect. Because pulmonary arterial hypertension and increased pulmonary artery resistance occur at an earlier stage, early closure of the sinus venosus ASD is indicated to avoid the consequences of chronic pulmonary hypertension. Sinus venosus ASD can be repaired only by surgical intervention. Intraoperative transesophageal echocardiography can be used to define the anatomy and physiology both pre‐ and postoperatively. Following median sternotomy, CPB is instituted using aortobicaval cannulation. Once the heart is arrested with antegrade blood cardioplegia, a vertical atriotomy is made from the atrial appendage to the pulmonary vein. This incision improves visualization of the defect, which can be repaired using autologous pericardium. Following de‐airing maneuvers, the patient is weaned from CPB. Echocardiography is performed to confirm unobstructed flow from the superior pulmonary vein to the left atrium and to rule out obstruction of superior caval vein flow from the pericardial patch. Patients should be placed on thromboprophylaxis for three months following repair to reduce the risk of postoperative embolic events. Ostium primum ASDs are part of the spectrum of AVSDs. With ostium primum ASD, the ventricular septum may be intact or there can be an associated restrictive (partial AVSD) or nonrestrictive inlet VSD (complete AVSD) [162]. In partial AVSD, the defect is adjacent to a common AV junction guarded by a common AV valve. In partial AVSD, the common five‐leaflet valve is divided into a trileaflet left and quadrileaflet right AV valve, which differ anatomically from the normal mitral and tricuspid valves. The trileaflet left AV valve has been described as a “cleft” mitral valve, as the commissure between the two bridging leaflets may resemble this. However, cleft mitral valve is a different anatomic entity to AVSD and the two should not be confused. The age of clinical presentation is variable depending on various hemodynamic factors. In fact, the hemodynamic consequences of partial AVSD are similar to those of secundum ASD, with varying degrees of left‐to‐right shunting depending on the physiology of the left and right ventricles, the presence and severity of left AV valve insufficiency, and the presence and size of a VSD. Many individuals present in the third and fourth decades of life with atrial arrhythmias or symptoms of congestive heart failure [163]. Atrial arrhythmias occur in 25% of patients by the age of 30 years and in 80% by the age of 45 years [164]
CHAPTER 47
Adult Congenital Heart Disease
General Considerations
Heart Failure
Pulmonary Arterial Hypertension
Arrhythmias
Rhythm disturbance
Associated lesions
Tachycardias
Wolff–Parkinson–White syndrome
Ebstein anomaly
Congenitally corrected transposition
Intra‐atrial reentrant tachycardia
(atrial flutter)
Postoperative Mustard
Postoperative Senning
Postoperative Fontan
Tetralogy of Fallot (TOF)
Other
Atrial fibrillation
Mitral valve disease
Aortic stenosis
TOF
Palliated single ventricle
Ventricular tachycardia
TOF
Aortic stenosis
Other
Bradycardias
Sinus node dysfunction
Postoperative Mustard
Postoperative Senning
Postoperative Fontan
Sinus venosus atrial septal defect (ASD)
Heterotaxy syndrome
Spontaneous atrioventricular (AV) block
AV septal defects
Congenitally corrected transposition
Surgically induced AV block
Ventricular septal defect (VSD) closure
Subaortic stenosis relief
AV valve replacement
Cyanosis
Hemostatic Dysfunction
Stroke
Renal Dysfunction
Metabolic Complications
Hemoptysis
Pregnancy and Heart Disease
Surgical Considerations
Resternotomy
Collateral Vessels
Blalock–Taussig–Thomas Shunt
Glenn Shunt
Pulmonary Artery Band
Anesthesia Considerations
Monitoring
Anesthesia in Specific Defects
Intracardiac Shunts
Tetralogy of Fallot
Postoperative Care
Respiratory Management
Cardiovascular Monitoring
Low Cardiac Output
Mechanical Assist Devices
Short‐Term Devices
Long‐Term Devices
Specific Congenital Heart Defects
Atrial Septal Defects
Secundum Atrial Septal Defects
Sinus Venosus Atrial Septal Defect
Ostium Primum Atrial Septal Defect
Stay updated, free articles. Join our Telegram channel
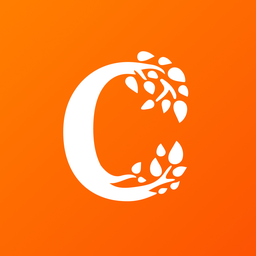
Full access? Get Clinical Tree
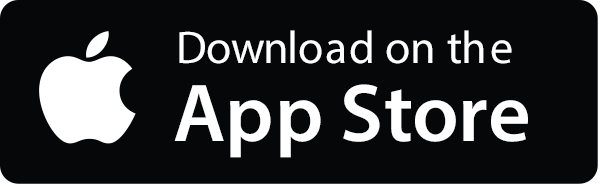
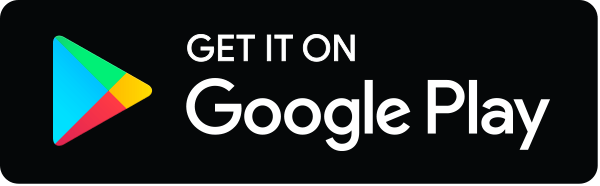