Hypoxemic Respiratory Failure
Hypoxemic respiratory failure has classically been defined as an arterial P o 2 of less than 60 mm Hg. It is distinguished from hypercapnic respiratory failure (P co 2 > 45 mm Hg), although the two conditions often coexist. There are a number of important qualifications to this definition that must be made. The threshold of 60 mm Hg is somewhat arbitrary and reflects the shape of the oxyhemoglobin dissociation curve, marking the arterial P o 2 below which the saturation of hemoglobin with oxygen falls precipitously in most people. An important caveat is that it is important whether hypoxemic respiratory failure is acute (over hours to days) or chronic (over weeks to months) as it has implications not just for diagnosis and treatment, but also for the physiologic adaptations to hypoxemia that develop over time. For example, an individual living at high altitude may have an arterial P o 2 less than 50 mm Hg because of a low inspired partial pressure of oxygen. Such an individual could well be asymptomatic because of acclimatization to that environment and would not be considered to have hypoxemic respiratory failure even if she had an arterial P o 2 of 45 mm Hg. It is also important to realize that this definition of hypoxemic respiratory failure (arterial P o 2 < 60 mm Hg) encompasses a broad spectrum of severity of illness. For example, both a patient on a general medical ward with community-acquired pneumonia and a mechanically ventilated patient on 100% oxygen in the critical care unit can fulfill the definition.
This definition of hypoxemic respiratory failure (based on the arterial P o 2 ) does not fully address the importance of oxygenation at the level of the tissues. Oxygen delivery to the tissues is the product of cardiac output and oxygen content. Oxygen content is critically dependent on the hemoglobin concentration and its saturation with oxygen; although the oxygen saturation depends on arterial P o 2 (as described by the oxyhemoglobin dissociation curve), the direct contribution of dissolved oxygen to blood oxygen content is very low under most conditions. In other words, in the anemic patient or in patients with very low cardiac output, tissue hypoxia may exist despite a seemingly adequate arterial P o 2 . Finally, as indicated earlier, both hypoxemic and hypercarbic respiratory failure may coexist. A patient may initially present with isolated hypoxemia yet, upon tiring, may develop hypercarbia. Analogously, hypoventilation can cause both hypercarbia and hypoxemia.
This chapter focuses on acute hypoxemic respiratory failure. In particular, much of our discussion pertains to the etiology and management of hypoxemia at the “severe” end of the spectrum. This is not to say that mild hypoxemia is not important; certainly the condition of a patient on the medical ward with pneumonia and mild hypoxemia can deteriorate and the patient would require intubation and mechanical ventilation.
Classification of Hypoxemia
The traditional approach to the causes of arterial hypoxemia classifies them into five pathophysiologic mechanisms: decreased inspired P o 2 , hypoventilation, impaired diffusion, ventilation-perfusion ( ) mismatch, and right-to-left shunt. Whereas hypoxemia from
mismatch is responsive to supplemental oxygen, hypoxemia from a right-to-left shunt is not. This physiologic approach is probably most useful in understanding how a particular disease causes hypoxemia, but is not usually very illuminating when trying to make a specific diagnosis, other than in situations in which a patient is hypoxemic due to profound hypercapnia. In a hospital setting, a decreased inspired P o 2 can usually be excluded, because most patients will be placed on supplemental oxygen. Hypoventilation can rapidly be excluded if the patient is not hypercapnic. Impaired diffusion by itself is not an important cause of acute hypoxemia, in that oxygen transfer across the alveolar-capillary membrane to red blood cells is usually perfusion-limited, not diffusion-limited. What this means is that there is usually ample time for diffusion of oxygen, even in the presence of intrinsic lung disease. In sum, most patients in the intensive care unit with acute hypoxemic respiratory failure have some combination of
mismatch and right-to-left shunt.
Another classification of acute hypoxemic respiratory failure is structural-anatomic ( Fig. 100-1 ). Causes of acute arterial hypoxemia can be classified based on whether the primary pathology is located in the air spaces, interstitium, heart and pulmonary vasculature, airways, or pleural space. Such an approach would quickly lead one to consider such causes as pulmonary edema or pneumonia, hypersensitivity pneumonitis, pulmonary embolism, bronchospasm, and pneumothorax. Although disorders involving other structures such as the central nervous system and respiratory muscles can lead to hypoxemia, these causes would be expected to have associated hypercarbia.

Clinical Features and Diagnostic Approach
The clinical features of acute hypoxemic respiratory failure vary based on the underlying cause. Assuming an intact drive to breathe and that the patient has not fatigued, the hypoxemic patient is usually tachypneic and tachycardic. Cyanosis of the lips or tongue (so-called central cyanosis) would indicate that the concentration of reduced (deoxygenated) hemoglobin is greater than 5 g/100 mL.
Given the extensive differential diagnosis of acute hypoxemic respiratory failure and the often urgent need for therapy, the clinician must be practical yet thoughtful. A basic history should be obtained to identify risk factors for cardiac dysfunction, pulmonary infection or aspiration, venous thromboembolism, or obstructive lung diseases. In the setting of trauma to the chest, pneumothorax, hemothorax, and pulmonary contusion must be considered. Further questions to identify less common causes of acute hypoxemic respiratory failure can be posed as appropriate. A focused physical examination of the cardiac and respiratory systems often can establish the presence or absence of congestive heart failure or a focal area of consolidation or effusion. Similarly, the diagnosis of a pneumothorax is more satisfying (and prompt) when made on physical examination rather than later, after chest imaging.
Implementation of therapy is simultaneous with the diagnostic workup. As always, this starts with the “ABCs” of airway, breathing, and circulation. Once the ABCs are assured, the patient should be given supplemental oxygen (if coexistent hypercapnia is present, care must be taken in the dose of supplemental oxygen) and intravenous access should be obtained. Continuous cardiac monitoring and pulse oximetry should be available.
The initial investigations are dictated by the findings on history and physical examination. However, all patients should have a chest radiograph, an electrocardiogram, and routine blood work, including a complete blood count with differential and serum chemistry. An arterial blood gas should be obtained and the alveolar-arterial P o 2 gradient should be calculated; a normal alveolar-arterial P o 2 gradient in the setting of arterial hypoxemia suggests hypoventilation as the sole cause of the hypoxemia. The blood gas is also useful for diagnosing other acid-base disturbances and hemoglobinopathies such as carbon monoxide poisoning. The need for further investigations, including bronchoscopy, computed tomographic angiography of the chest, and echocardiography, depends on the results of the initial assessment. A completely normal chest radiograph in the setting of hypoxemic respiratory failure narrows the differential diagnosis substantially. In this uncommon circumstance, the clinician should consider pulmonary embolism and right-to-left shunts (i.e., intracardiac or pulmonary arteriovenous malformations) as possibilities. A more common scenario is that the chest radiograph of a patient with pneumonia can appear surprisingly normal (or may show only a “small” opacity) due to concomitant intravascular volume depletion. Once intravascular volume has been restored, the extent of the opacity can be appreciated.
Causes of Acute Respiratory Failure
In a large multicenter international prospective cohort study of patients requiring mechanical ventilation, the most common reported causes of acute respiratory failure were postoperative respiratory failure, pneumonia, congestive heart failure, sepsis, and trauma. In a small prospective cohort study that included 41 patients with hypoxemic respiratory failure, chronic obstructive pulmonary disease and pneumonia were the most common causes. Other data from small randomized controlled trials of noninvasive ventilation identified congestive heart failure, pneumonia, trauma, acute respiratory distress syndrome, and mucous plugging as the most common causes of respiratory failure. However, in these studies, patients with certain diseases were excluded, including those with chronic obstructive pulmonary disease and asthma, limiting the ability to generalize from these findings. Indeed, in the one randomized study that specifically enrolled patients with acute hypoxemic respiratory failure, only patients with bilateral lung opacities on chest radiography were included.
For a detailed discussion of many of the specific causes of acute hypoxemic respiratory failure (e.g., pneumonia), refer to the appropriate chapters in this book. The remainder of this chapter focuses on a particular subtype of acute hypoxemic respiratory failure, known as acute respiratory distress syndrome (ARDS).
Acute Respiratory Distress Syndrome
Diagnosis and Epidemiology
Diagnosis
ARDS is characterized by noncardiogenic pulmonary edema, lung inflammation, hypoxemia, and decreased lung compliance. Unlike some disorders (e.g., coronary artery disease), ARDS, as its name suggests, is a syndrome, reflecting a constellation of clinical and physiologic observations thought to represent a common pathology. In coronary artery disease, narrowing of the coronary vasculature and disruption of an unstable atherosclerotic plaque are known to underpin the symptoms of angina and unstable angina, respectively. A diagnostic “gold standard,” namely coronary angiography, exists. In contrast, the pathogenesis of ARDS remains elusive and there is no gold standard diagnostic test. The heterogeneity of the clinical conditions associated with ARDS (discussed later) would be consistent with the possibility that ARDS is in fact a collection of different diseases that have not yet been separately identified. These problems necessarily permeate any discussion or research into ARDS, and this chapter is no exception.
The first description of ARDS appeared in a remarkable case series reported in 1967. Ashbaugh and colleagues described 12 patients ranging in age from 11 to 48 years who presented with respiratory distress, hypoxemic respiratory failure, and patchy bilateral opacities on chest radiographs ( Fig. 100-2 ). Most of the cases were preceded by severe trauma or viral infection and the onset of symptoms was relatively rapid, with most patients developing respiratory distress within 48 to72 hours of the beginning of their illness. Many patients required positive pressure ventilation and exhibited low respiratory system compliance, and some experienced improvement in oxygenation with the application of positive end-expiratory pressure (PEEP). This syndrome was initially termed the adult respiratory distress syndrome to distinguish it from the respiratory distress syndrome seen in infants. Subsequently, recognizing that the syndrome can also develop in children, it was renamed the acute respiratory distress syndrome.

The cases described by Ashbaugh and colleagues generated interest and research into ARDS. Unfortunately, the lack of specific diagnostic criteria and an understanding of the pathogenesis of the disorder made it difficult to undertake research and to compare studies. In 1988, a formalized and expanded definition of ARDS was proposed that consisted of three parts: (1) determining whether the illness was acute or chronic, (2) determining whether there were any associated risk factors or medical conditions (e.g., sepsis), and (3) assigning points based on the severity of pulmonary dysfunction (the Lung Injury Score), as measured by the degree of hypoxemia, the level of PEEP required, the respiratory system compliance, and the degree of radiographic abnormality. An average score was calculated and a final value greater than 2.5 was used to diagnose ARDS. One advantage of this system was its description of associated medical conditions, which might be pertinent to the etiology of ARDS. Because ARDS from different etiologies might reflect different pathogenesis and perhaps confer a different response to therapy, it was thought that knowing the cause of ARDS might be important in the conduct and comparison of studies. The definition, however, made no attempt to exclude cardiogenic pulmonary edema; it was assumed that clinicians would do so automatically.
In 1994, a consensus conference of American and European investigators published their definition of ARDS, which was widely adopted. Aiming for simplicity, ARDS was defined as a syndrome of acute onset, with bilateral opacities on chest radiography consistent with pulmonary edema, pulmonary artery occlusion pressure of 18 mm Hg or less (or absence of clinical evidence of left atrial hypertension), and hypoxemia as measured by the ratio of the arterial partial pressure of oxygen (arterial P o 2 ) to the fraction of oxygen inspired (F io 2 ). Recognizing that there was a spectrum of severity of the disease, the consensus panel recommended that arterial P o 2 /F io 2 ratio ≤ 300 would define an entity termed acute lung injury (ALI). ARDS was the most severe form of ALI and was diagnosed when arterial P o 2 /F io 2 ≤ 200. The simplicity of the definition led to its general acceptance by clinicians and its incorporation into clinical research. At the same time, such a straightforward definition could not take into account the heterogeneity of the disease or the ambiguity of clinical practice.
For example, the requirement for bilateral opacities on chest radiograph is open to interpretation. One study presented a random series of chest radiographs from intubated hypoxemic patients (arterial P o 2 /F io 2 < 300) to an international group of expert clinicians, the majority of whom conduct clinical research on ARDS. The clinicians were asked to examine each radiograph and to decide whether it fulfilled the American-European Consensus Conference (AECC) definition of ARDS (bilateral opacities consistent with pulmonary edema). This select group of physicians demonstrated only moderate agreement (kappa statistic 0.55) in their classification of the radiographs; when the percentage of radiographs deemed to be consistent with ARDS by each physician was examined, the percentages were evenly distributed from a low of 36% to a high of 71%. Another larger study on the issue also demonstrated only moderate agreement when two physicians were asked to rate radiographs on the presence or absence of diffuse bilateral opacities consistent with ARDS. However, with prior training and discussion, the agreement between an intensivist and a radiologist in the rating of the films was excellent.
The AECC definition has also been criticized for not taking the level of PEEP into consideration. Clinicians have long appreciated that applying PEEP can improve oxygenation, an observation that was noted in the first description of ARDS. This implies that the arterial P o 2 /F io 2 will change with changes in the level of PEEP; indeed, a patient who meets the AECC criteria for ARDS may no longer meet them once PEEP has been increased. It has also been shown that patients meeting the AECC definition for ARDS can be stratified based on their response to standard ventilator settings 24 hours later; many of the patients at that point have a lesser degree of hypoxemia and a significantly lower mortality rate. Thus, the AECC definition, while simple to use, encompasses a heterogeneous group of patients.
In 2012, the so-called Berlin definition of ARDS was developed to address some of these limitations ( Table 100-1 ). The degree of hypoxemia was stratified into mild, moderate, and severe based on the arterial P o 2 /F io 2 ratio and a requirement for a PEEP level of 5 cm H 2 O or greater was included (whether applied by endotracheal tube or, in the setting of mild disease, by noninvasive ventilation). The term ALI was eliminated (but is used as needed in this chapter to help readers interpret older literature). Given that the use of pulmonary artery catheters has fallen off dramatically due to a demonstrated lack of benefit, the need for a pulmonary arterial wedge pressure measurement was eliminated and instead it was recommended that objective testing (e.g., echocardiography) be performed to exclude cardiogenic edema if no risk factor for ARDS could be identified. “Acute” was explicitly defined as ARDS developing within 1 week of a known risk factor. Although considerable effort went into its design, when compared with the AECC criteria, the Berlin definition is only slightly better at predicting mortality attributable to ARDS.
Criterion | Definition |
---|---|
Timing | Within 1 week of a known precipitant, or new/worsening respiratory symptoms |
Chest imaging (chest radiograph or CT scan) | Bilateral opacities—not fully explained by effusions, lobar/lung collapse, or nodules |
Origin of edema | Respiratory failure not fully explained by cardiac failure or fluid overload If no risk factor for ARDS is present, need objective assessment (e.g., echocardiogram) to exclude hydrostatic edema |
Oxygenation | |
Mild | 200 mm Hg < arterial P o 2 /F io 2 ≤ 300 mm Hg with PEEP or CPAP ≥ 5 cm H 2 O |
Moderate | 100 mm Hg < arterial P o 2 /F io 2 ≤ 200 mm Hg with PEEP ≥ 5 cm H 2 O |
Severe | Arterial P o 2 /F io 2 ≤ 100 mm Hg with PEEP ≥ 5 cm H 2 O |
Two additional points should be made about the diagnosis of ARDS. First, while physicians properly think of ARDS as being distinct from cardiogenic pulmonary edema, it is important to acknowledge that many patients with ARDS will have left atrial hypertension during the course of their illness. Second, despite the increasing use of B-type natriuretic peptides as diagnostic tools for acute congestive heart failure, their ability to distinguish ARDS from cardiogenic pulmonary edema is unclear.
Incidence
Given the multitude of definitions and the difficulty in making a diagnosis of ARDS, it is not surprising that determining the incidence of the disease has been challenging. The reported incidence of ARDS has ranged from 75 per 100,000 population to as low as 1.5 per 100,000 in Europe; the European numbers appear fairly constant over time. Many older studies did not use the AECC definition and did not include ALI; the Berlin definition is too recent to have been widely studied. The incidence of ALI has been estimated to be 78.9 cases per 100,000 person-years. One study calculated an incidence of ALI of 22 cases per 100,000 using data from a large prospective trial of ARDS and from the American Hospital Association. One of the methodologic strengths of the study was its inclusion of cases of ALI over a 3-year period, thereby decreasing the impact of seasonal variability. The heterogeneity in the literature of the estimates of incidence may reflect differences in the methodology of the various studies, but may also reflect true variation. Regional differences in genetic or environmental factors and specific disease-associations such as cardiopulmonary bypass or lung transplant may account for some of the regional variability in the incidence of ALI.
Risk Factors
Sepsis, aspiration of gastric contents, and multiple transfusions (>15 units/24 hours in one study) are associated with the highest risk for development of ARDS. In particular, ARDS develops in almost 40% of patients with sepsis. The presence of more than one presumed risk factor for ARDS may increase the incidence of ARDS, although the sample sizes of these subsets have been quite small. ARDS has been seen to develop in more than one third of patients receiving massive blood transfusion and in one fourth of patients with multiple trauma (one or more pulmonary contusions, multiple fractures, multiple transfusions).
One of the predisposing factors for the development of ARDS is a history of chronic alcoholism (relative risk, 2.0; 95% confidence interval, 1.3–2.9). This relationship has been shown to remain significant after adjustment for gender, at-risk diagnosis, and severity of illness. Among those in whom ARDS developed, patients with a history of alcoholism had a higher mortality rate than those without such history (odds ratio, 6.3; 95% confidence interval, 2.2–20.4). The mechanisms of this association are unknown.
The same researchers examined whether patients with diabetes might be protected against development of ARDS, the rationale being that hyperglycemia is known to impair neutrophil function, and neutrophils are thought to be central to the pathogenesis of ARDS (see Pathogenesis ). More than 100 patients admitted to the intensive care unit with septic shock were followed prospectively for the development of ARDS. Over a 2-year period, diabetes was associated with a significantly decreased risk of ARDS (relative risk, 0.53; 95% confidence interval, 0.28–0.98), which persisted after adjustment for the source of sepsis and other potential confounders. Interestingly, there was no difference in mortality between patients with ARDS who did and did not have diabetes.
A systematic overview of studies examining potential risk factors for ARDS concluded that the strongest evidence of a causal relationship was for sepsis, trauma, multiple transfusions, aspiration of gastric contents, pulmonary contusion, pneumonia, and smoke inhalation. More recently, active or passive exposure to cigarette smoke has been associated with the development of ARDS after trauma.
The heterogeneity of risk factors for ARDS is remarkable. At the AECC, investigators divided the known risk factors into those thought to cause direct lung injury (e.g., pneumonia) and those in which the mechanism of lung injury was thought to be indirect (e.g., pancreatitis) ( Table 100-2 ). While conceptually attractive, this classification scheme may not reflect underlying differences in the mechanisms, severity, or outcome of lung injury. This issue is discussed in more detail in the section on outcomes.
Direct injury | Indirect injury |
---|---|
Pneumonia | Sepsis |
Aspiration | Major trauma |
Pulmonary contusion | Multiple blood transfusions |
Toxic inhalation | Pancreatitis |
Near-drowning | Cardiopulmonary bypass |
Reperfusion injury (e.g., after lung transplantation) | Drug overdose |
Adverse effect of medication |
Etiology and Pathogenesis
Overview of Pathophysiology
In the past, ARDS was also called noncardiogenic pulmonary edema, a descriptive term that nonetheless reflected what was known of the pathogenesis of the disorder. Unlike congestive heart failure, in which elevated left-sided cardiac pressures cause hydrostatic pulmonary edema, in ARDS, the edema fluid that fills the alveoli is exudative in origin. In other words, the alveolar-capillary barrier exhibits increased permeability, allowing for the leakage of protein-rich fluid into the air spaces. Alveolar filling leads to decreased respiratory system compliance as well as right-to-left shunting and profound hypoxemia. Although arterial P co 2 is generally within the normal range, dead space ventilation is significantly increased, as demonstrated by elevated minute ventilation. Pulmonary hypertension is also commonly observed in ARDS, and a number of mechanisms have been proposed including hypoxic vasoconstriction, intravascular fibrin deposition in the pulmonary capillaries, and compression of blood vessels by the positive pressure ventilation used to treat the disorder. This section reviews the pathology of ARDS and discusses current theories regarding its pathogenesis. Much of the research into ARDS has focused on determining the basis for the increase in alveolar-capillary permeability.
Pathology
The pathologic features of ARDS have classically been described using three overlapping and sequential stages. In the first or exudative phase of lung injury, the pathologic findings have been termed diffuse alveolar damage. There are hyaline membranes lining the alveolar walls and protein-rich edema fluid in the alveolar spaces, as well as epithelial disruption and infiltration of the interstitium and air spaces with neutrophils. Areas of hemorrhage and macrophages can also be found in the alveoli. This phase, which is thought to last for approximately 5 to 7 days, is followed in some patients by the so-called proliferative phase. At this point, hyaline membranes are reorganized and fibrosis begins to be observed. Obliteration of pulmonary capillaries and deposition of interstitial and alveolar collagen may be observed, along with a decrease in the number of neutrophils and the extent of pulmonary edema. This proliferative stage has traditionally been described as being followed by a fibrotic phase, essentially emphasizing the appearance of pulmonary fibrosis in a subset of patients with persistent (i.e., >2 weeks) ARDS. More recently, it has been realized that areas of fibrosis may actually develop sooner than usually appreciated: elevated levels of N-terminal procollagen peptide III, thought to represent collagen synthesis, can be detected in bronchoalveolar lavage fluid of patients with ARDS as early as 24 hours into the course of the illness. In addition, the bronchoalveolar lavage fluid from these patients has been shown to stimulate cultured fibroblasts to proliferate. These and other observations have led some investigators to hypothesize that fibroproliferation may be initiated simultaneously with (rather than after) inflammatory lung injury.
Alveolar-Capillary Membrane
If ARDS is a disorder of increased alveolar-capillary permeability, it stands to reason that the pulmonary microvascular endothelium or the alveolar epithelium (or both) must be involved in its pathophysiology. Damage to the alveolar epithelium is thought to be a key event. Once ARDS has begun, the importance of the alveolar epithelium is also clear: Type II pneumocytes must differentiate into type I cells to help cover the denuded epithelial surface, while both type I and II cells express the Na + ,K + -ATPase, which is thought to be important in clearance of edema fluid (discussed later). Injury to type II pneumocytes impairs the production and metabolism of surfactant. In addition to damage to the alveolar epithelium, loss of lung endothelial barrier integrity is both necessary and sufficient for the development of ARDS. How the alveolar-capillary membrane is damaged is not known for certain, although mediators of epithelial and endothelial apoptosis and neutrophils are suspected of playing a role (see later).
Surfactant.
Surfactant is a complex mixture of phospholipids and surfactant proteins that reduces alveolar surface tension. Many researchers have described alterations to the surfactant obtained from patients with ARDS. There are decreased amounts of surfactant-related protein and decreased dipalmitoylphosphatidylcholine and phosphatidylglycerol in the surfactant of patients with ARDS. The proportion of large (active) to small (inactive) aggregates of surfactant is diminished, due to both decreased production of surfactant and increased conversion of the large to small forms. In addition, plasma proteins that have leaked through the alveolar-capillary barrier may interfere with surfactant function. For example, damage to surfactant protein A has been demonstrated in patients with ARDS. The impairment in surfactant function observed in ARDS would theoretically predispose alveolar units to collapse. In addition, surfactant proteins have been found to have antimicrobial properties, although whether the loss of these properties is relevant to the pathogenesis of ARDS is not known. Indeed, despite the litany of abnormalities in surfactant that have been described, the extent to which these alterations contribute to the pathogenesis of ARDS remains controversial: unlike the neonatal respiratory distress syndrome, in which a deficiency of surfactant underlies the pathophysiology of the disease, it is possible that the abnormalities of surfactant in ARDS are the result rather than the cause of altered physiology. Indeed, four large randomized controlled trials of exogenous surfactant supplementation in ARDS failed to demonstrate any improvement in mortality or requirement for mechanical ventilation (see Treatment). Only one trial, using calfactant (a natural surfactant, distinct from the synthetic surfactants used in previous trials) in pediatric patients with ARDS, has shown any benefit ; whether this is due to the unique surfactant used in this study or to differences between the patients enrolled in this trial and the negative trials is unknown.
Neutrophils and Other Inflammatory Mediators
Histologically, one of the hallmarks of ARDS is the accumulation of neutrophils in the microvasculature of the lung. As key players in innate immunity, neutrophils can generate an impressive array of cytotoxic compounds. These include reactive oxygen species, cationic peptides (e.g., defensins), eicosanoids, and proteolytic enzymes such as leukocyte elastase. In addition, once activated, neutrophils release growth factors and cytokines (e.g., tumor necrosis factor [TNF] – α and interleukin [IL]-1β) that may enhance the inflammatory response. Given this destructive potential, it has long been theorized that neutrophils may be central to the pathogenesis of ARDS ( Fig. 100-3 ). There is an abundance of clinical and preclinical data to support this hypothesis. For example, in humans with ARDS following sepsis, bronchoalveolar lavage neutrophilia is associated with a poor prognosis. Neutrophil-depleted mice exposed to hyperoxia develop less lung injury than normal mice. Similarly, in a hamster model of ARDS induced by endotoxin inhalation, the administration of a specific inhibitor of neutrophil elastase (even hours after administration of endotoxin) prevented the development of lung injury.

If neutrophils are involved in the pathogenesis of ARDS, some defect in their regulation must be invoked. For example, in an uncomplicated bacterial pneumonia, the pulmonary inflammatory response is limited by counter-regulatory processes that prevent tissue damage. Whether this regulation is ineffective or insufficient in ARDS is a fascinating and unanswered question.
One of the earliest manifestations of ARDS, even before hypoxemia, is a transient leukopenia due to sequestration of neutrophils in the lung microvasculature. The average pulmonary capillary is smaller than the average neutrophil, and neutrophils therefore have to deform to pass through the microvasculature. Activated neutrophils “stiffen” and cannot negotiate narrow capillary segments. Inhibitors of actin polymerization can abrogate this stiffening, implicating a change in the actin cytoskeleton in neutrophil sequestration in the lung. Neutrophil sequestration also involves interactions between molecules on the surface of the neutrophil and the lung capillary endothelium. For example, in rabbits, blocking the adhesion molecule L-selectin on the surface of the neutrophil prevented neutrophil sequestration in alveolar capillaries induced by exposure to endotoxin.
After the initial sequestration, neutrophils must translocate (via diapedesis) across the alveolar-capillary barrier to the alveolar space. The determinants of this seemingly simple movement remain incompletely understood. Integrins on the surface of the neutrophil are thought to mediate emigration of neutrophils from the pulmonary circulation in response to some, but not all, inflammatory stimuli. Proteases secreted by the alveolar epithelium, in concert with regional cytokine production and glycosaminoglycans on the epithelial surface, may act together to form a chemotactic gradient for neutrophils to follow.
Many studies are focusing on the mechanisms of neutrophil activation whereas others are focusing on the interaction between platelets and neutrophils and how mutual activation may contribute to ARDS or sepsis. Other studies have looked at various components of intracellular signal transduction pathways, such as kinases (enzymes that phosphorylate substrates) and transcription factors. For example, one such kinase is the p38 mitogen-activated protein kinase, which is activated when cells are stimulated with lipopolysaccharide (LPS). Activation of this kinase stimulates TNF-α production and macrophage inflammatory protein-2 release (a chemotactic factor for macrophages). Interestingly, inhibition of p38 mitogen-activated protein kinase in mice, even hours after the mice are exposed to aerosolized LPS, attenuates neutrophil chemotaxis and migration from the lung microvasculature into alveoli. Another kinase often discussed in the context of inflammation is phosphatidylinositol 3-kinase. This enzyme phosphorylates phosphatidylinositol, a lipid-derived second messenger whose phosphorylated forms have been implicated in a myriad of intracellular signaling events. Phosphatidylinositol 3-kinase γ is preferentially activated in neutrophils exposed to IL-8 or fMLP (a bacterial-derived peptide). Despite exposure to intraperitoneal endotoxin, phosphatidylinositol 3-kinase γ knockout mice displayed decreased neutrophil accumulation, cytokine production, and lung injury compared to control mice. The neutrophils in the lung of the knockout mice also demonstrated diminished activation of NF-κB, an important transcription factor known to mediate the up-regulation of numerous cytokines and proinflammatory mediators.
There are many potential mechanisms by which activated neutrophils might mediate ALI. In addition to secreting cytokines and growth factors that might stimulate the local and systemic inflammatory response, neutrophils release defensins and generate reactive oxygen species that can mediate tissue damage. In animal studies, inhibition of the assembly of NADPH oxidase (the major source of reactive oxygen species) by apocynin has been shown to attenuate sepsis-induced lung injury. The nitric oxide synthase (NOS) pathway has also been implicated in mediating lung injury; knockout mice lacking the gene for inducible NOS developed less severe lung injury than wild-type animals upon injection with LPS.
In addition, neutrophils contain proteolytic enzymes that may be involved in the pathogenesis of ALI. In particular, both neutrophil elastase and metalloproteinases have been extensively studied. Because of its ability to degrade multiple substrates including growth factors and cytokines, neutrophil elastase may be involved in regulation of the inflammatory response. It has also been shown to degrade epithelial and endothelial cadherins, proteins that are major components of adherens junctions, which hold cells together. It is possible that elastase-mediated destruction of cadherin could predispose to alveolar flooding. Despite its potential to cause unwanted tissue damage, neutrophil elastase (NE) is likely crucial to host defense. In NE-deficient mice, the administration of intraperitoneal Klebsiella pneumoniae causes 100% mortality within 48 hours, whereas mortality in normal mice was only 50%. However, NE-deficient mice are paradoxically resistant to normally lethal doses of LPS. Furthermore, mice that lack both NE and another neutrophil protease (cathepsin G) are protected against alveolar damage induced by endotoxic shock. These apparently contradictory results suggest that NE, while important in a regulated inflammatory response, may nonetheless participate in inflammatory injury under certain circumstances. In numerous studies of multiple different experimental models in multiple species of animals, NE has been shown to play an important role in the pathogenesis of ALI. Whether it is as important in the development of ARDS in humans remains unanswered. A clinical trial of a leukocyte elastase inhibitor in ARDS was halted because preliminary analysis suggested lack of efficacy.
Metalloproteinases may also be important mediators of leukocyte-mediated lung injury. Elevated concentrations of the matrix metalloproteinases gelatinase A and B have been found in the epithelial lining fluid of patients with ARDS. Mice lacking the genes for gelatinase B or stromelysin 1 had less severe injury in an animal model of ALI. As mentioned earlier, matrilysin (matrix metalloproteinase 7) has been shown to regulate the formation of a chemotactic gradient and the transmigration of neutrophils across the alveolar epithelium in a mouse model of ALI. Finally, administration of an inhibitor of elastase and metalloproteinases (chemically modified tetracycline-3) attenuated ALI after cardiopulmonary bypass in pigs.
Despite the emphasis on neutrophils as potentially causative for lung injury, it is important to note that ARDS has certainly been described in patients with profound neutropenia. Indeed, it is possible that the neutrophil infiltration observed in some cases of ARDS is adaptive (i.e., a physiologic response to a primary injury) rather than destructive. At present, however, it is generally believed that neutrophils are a causative factor in the development of most cases of ARDS. Comparatively little is known about the mechanisms of neutrophil-independent ARDS and this must be a goal of future investigations.
Inflammation and Coagulation
Because one of the most common precipitants for ARDS is sepsis, it is hoped that therapies for sepsis may either prevent or improve the outcome of ARDS. Our current concept of sepsis is that there is an initial inflammatory state, with up-regulation of inflammatory cytokines such as TNF-α and IL1β and recruitment and activation of inflammatory cells such as neutrophils. This early stage of inflammation is followed by a relatively depressed immunity, in which the patient is vulnerable to nosocomial infections. The theory that dysregulation of the inflammatory response is responsible for sepsis has led to the search for agents that would selectively block components of the inflammatory pathway. It has also been appreciated that there are important connections between the molecular cascades that regulate both inflammation and coagulation; for example, TNF-α causes an increase in thrombin and fibrin formation, while fibrin fragments themselves are known to be chemotactic for neutrophils. TNF-α increases tissue factor expression on endothelium and inhibits fibrinolysis, both of which favor fibrin formation. Activated protein C (APC), an endogenous anticoagulant, has direct and indirect anti-inflammatory effects, which include reducing levels of IL-6 and attenuating neutrophil activation in sepsis. Because sepsis is the most common cause of ARDS and because abnormalities of coagulation are very common in sepsis, investigators have wondered whether altered coagulation is involved in the genesis of ARDS. How this might happen is largely speculative, but intraalveolar, interstitial, and intravascular fibrin deposition have all been observed in patients with ARDS. Indeed, fibrin is a major component of hyaline membranes. Intraalveolar fibrin has been postulated to serve as a nidus for fibroblast proliferation and potentially, as ALI resolves, as a stimulus for pulmonary fibrosis. Fibrin might contribute as well to ongoing lung injury through its chemotactic properties, and intravascular fibrin deposition, as in microthrombi, might contribute to the elevated pulmonary vascular pressures observed in ARDS.
Interest in the role of coagulation in the pathogenesis of ARDS was buttressed in part by observations in animal studies that anticoagulation can attenuate the severity of sepsis-induced lung injury, although studies in humans have been disappointing. Indeed, a small randomized controlled trial of APC in patients with ALI was stopped prematurely due to lack of efficacy, and APC was pulled from the market after a large multicenter trial showed it provided no mortality benefit in patients with septic shock.
Other molecular determinants of inflammation continue to be elucidated. Some data have implicated transforming growth factor-β in an animal model of ALI. In one study, transforming growth factor-β was postulated to cause increased alveolar epithelial cell permeability by depleting intracellular glutathione levels. Other investigators have attempted to improve the endogenous counter-inflammatory response; in one study, the gene for heat shock protein 70 was ligated to an adenoviral promoter, and the resultant recombinant construct was administered into the lungs of rats in which ARDS had been induced by cecal ligation and perforation. Administration of the heat shock protein 70 construct increased heat shock protein 70 expression specifically in the lung; remarkably, this increased expression was associated with a significant reduction in pulmonary edema and inflammation and even mortality. Indeed, mice deficient in heat shock protein 70 showed increased mortality and lung injury after cecal ligation and perforation. The mechanisms underlying this effect are not clear, but may involve suppression of the proinflammatory transcription factor NF-κB or decreased lung parenchymal apoptosis.
Na + and Water.
Na + channels at the apical surface of alveolar epithelial cells mediate intracellular Na + uptake from the alveoli. This creates an osmotic gradient that causes alveolar fluid to follow, thereby allowing for the clearance of pulmonary edema. Na + ,K + -ATPases in the basolateral membrane of the epithelial cell exchange intracellular Na + for extracellular K + , maintaining the intracellular sodium concentration low enough for the apical Na + resorption to continue. This process allows for the net removal of Na + and fluid from the alveolar space, and can be accelerated (at least experimentally) by catecholamines.
Dysfunction of either the apical Na + channels or the basolateral Na + ,K + -ATPases (or both) have been postulated to be involved in the pathogenesis of ARDS. For example, it is known that hypoxia decreases epithelial Na + reabsorption by impairing the expression of subunits of the epithelial Na + channel. Hypoxia also decreases the activity of both the apical Na + channel and the Na + ,K + -ATPase. In an animal model of hemorrhagic shock-induced lung injury, the normal up-regulation of alveolar fluid reabsorption in response to catecholamines was absent. This defect was attributed to an increase in nitric oxide (NO) in the lung, possibly due to an effect on β-adrenergic receptor signaling. In a study of patients with ARDS, alveolar fluid clearance was calculated from the change in alveolar fluid protein concentrations. When patients were stratified by their rate of fluid clearance, those with the highest rates had the shortest duration of mechanical ventilation and the lowest mortality. In a model of ventilator-induced lung injury (VILI) in rats, overexpression of the Na + ,K + -ATPase improved the clearance of lung liquid. However, attempts to increase alveolar fluid clearance in a clinical setting (e.g., by β-adrenergic agonists) have so far been disappointing ; further details are provided later under Novel Therapies (see also Chapter 9 ).
Angiopoietins.
Angiopoietins are peptides involved in embryonic vascular development. Of the four angiopoietins that have been identified, angiopoietin 1 and angiopoietin 2 (ANGPT1 and ANGPT2, respectively) are the best described. ANGPT1 is expressed by numerous cell types while ANGPT2 is mostly limited to endothelial cells. Both act on the TIE2 receptor tyrosine kinase that is found predominantly on endothelial cells and hematopoietic stem cells. A number of observations have generated interest in the potential role of these proteins in the pathophysiology of ARDS. First, elevated levels of ANGPT2 have been described in patients with sepsis and ARDS and its administration to mice causes pulmonary vascular leak. The effect of ANGPT2 in disrupting endothelial cell integrity could be reversed in vitro by ANGPT1. Second, overexpression of ANGPT1 was protective against both endotoxin-induced septic shock and ALI in mice ; a similar benefit was observed using a synthetic peptide agonist of the TIE2 receptor. While the cellular mechanism of these effects remains uncertain and is the subject of intense investigation, the data to date emphasize the important role for endothelial cells in the development of and recovery from ARDS.
Ventilator-Induced Lung Injury
Although mechanical ventilation for ARDS may be lifesaving, there is abundant preclinical and clinical evidence that it can also be harmful. While it was quickly recognized that excessive airway pressure could lead to barotrauma ( Fig. 100-4 ), including pneumothorax, pneumomediastinum and subcutaneous emphysema, attention has since turned to subtler but more common manifestations of lung injury related to mechanical ventilation. Mechanical ventilation can induce pulmonary edema by causing increases in both epithelial and endothelial permeability. Indeed 40 years ago Webb and Tierney demonstrated that ventilation of rats with high peak airway pressures could lead to severe pulmonary edema, and more than 20 years ago, investigators noted that mechanical ventilation could produce a form of increased permeability pulmonary edema remarkably similar to ARDS. Now, the accumulated evidence suggests that certain mechanical ventilation strategies may aggravate if not induce ARDS in some patients.

A major mechanism causing VILI is overdistention of lung units, rather than the absolute airway pressure per se. Normal rats ventilated with high airway pressure due to a high tidal volume developed increased permeability, whereas rats ventilated with smaller tidal volumes, but with the same end-inspiratory pressure (obtained by strapping the chest walls of the rats) did not develop increased permeability. Rats were also ventilated with low airway pressure using negative inspiratory pressure (applied at the chest wall) and high tidal volumes. Their results demonstrated that the rats ventilated with high tidal volumes had significantly more edema than others. In particular, the negative (low) pressure and high tidal volume group had the worst edema. These important observations, confirmed in other species, led to the appreciation that large tidal volumes, rather than high airway pressures per se, are an important determinant of ventilator-induced pulmonary edema. The term volutrauma was created to recognize this fact.
The repetitive opening and closing of terminal lung units associated with mechanical ventilation is also considered to be detrimental. The mechanism of this injury, which has been termed atelectrauma, is thought to be the high shear stresses generated at the interface of collapsed and aerated tissue when a collapsed airway is reopened. Theoretically, PEEP should be helpful in minimizing this injury by keeping the lung recruited and promoting greater lung homogeneity; any such advantage of PEEP would have to be balanced against the potential harm caused by a potentially greater overdistention of lung units due to the higher PEEP levels (see ).
It is important to point out that patients with ARDS may be especially vulnerable to VILI because of the heterogeneous nature of the pulmonary parenchymal injury. On computed tomography scans of the lungs, normal-appearing lung and densely consolidated injured lung are both seen; as a consequence, there are marked regional differences in lung compliance ( Fig. 100-5 ). A tidal volume designed to inflate an entire lung would preferentially inflate the normal-appearing areas, potentially leading to overdistention and volutrauma. Patients with ARDS may similarly be more vulnerable to atelectrauma. Although some evidence suggests that normal lungs can tolerate at least short periods of cyclic opening and closing of airways from mechanical ventilation, injured lungs, such as in ARDS, would be exposed to much higher shear stresses and would not be expected to fare as well.

Over the past 15 years, there has been a growing appreciation that VILI is not only a mechanical injury, but also reflects an underlying complex cellular and molecular response. The term biotrauma has been coined to emphasize this change in thinking. In our understanding of VILI, one of the most significant advances made is that mechanical ventilation per se can induce both a local and systemic inflammatory response. In animal studies of ex vivo lungs, it has been shown that, compared to a control ventilation strategy with moderate tidal volumes and PEEP, ventilation either with high tidal volumes or with zero PEEP causes elevations in lung lavage levels of inflammatory cytokines. Lungs ventilated both with high tidal volumes and with zero PEEP had a synergistic elevation in cytokine levels. This inflammatory response to an injurious ventilation strategy has also been shown to extend beyond the lung. In an acid aspiration rat model of lung injury, a ventilatory strategy using high tidal volumes and zero PEEP was associated with an increase in blood levels of various cytokines; this was not observed in groups ventilated with a small tidal volume or in the group ventilated with the large tidal volume but higher PEEP levels.
Most importantly, similar observations have been made in humans with ARDS. In one study, patients were randomized to “lung-protective” ventilation, in which the tidal volume was set to avoid overdistention and PEEP was set above the lower inflection point of the pressure volume curve. The control group received a strategy that would have been considered conventional ventilation at the time. While alveolar-lavage and plasma cytokine levels declined in the lung-protective group, they rose significantly in the control group. Post hoc analyses revealed a significantly higher number of ventilator-free days in the lung-protective group than in controls and significant correlations between the development of multisystem organ failure and plasma cytokines. The impact of ventilator strategy on the development of biotrauma can be remarkably quick. Within 1 hour after switching patients from a protective ventilatory strategy, Stuber and colleagues found an increase in a number of proinflammatory cytokines in the lungs and plasma of patients with ARDS.
Mortality and Complications
As discussed in the section on outcomes, patients with ARDS often die of the systemic inflammatory response syndrome and multiorgan dysfunction. Hence, the observation that mechanical ventilation can influence pulmonary and systemic cytokine levels is intriguing. Taken together, these findings suggest that mechanical ventilation has the potential not only to injure the lungs, but also to lead to a loss of compartmentalization of the pulmonary inflammatory response. Systemic dissemination of this response could be associated with the development of systemic inflammatory response syndrome and potentially with multisystem organ failure ( Fig. 100-6 ). In one study, injurious mechanical ventilation in rats not only caused elevated plasma and lung cytokine levels, but also caused increased renal epithelial cell apoptosis and associated renal dysfunction. Furthermore, pretreatment with either IL-10 (an anti-inflammatory cytokine) or IL-22 (a member of the IL-10 family that has immunoregulatory and tissue protective properties) reduced lung injury and reduced mortality in animal models of VILI.

Finally, patients with ARDS often require very high inspired fractions of oxygen. The toxic effects of hyperoxia on the lung have been well described, and the histologic appearance mirrors that of human ARDS. Oxygen toxicity is thought to be mediated by the formation of both reactive oxygen and nitrogen species, which can damage tissues by a multitude of mechanisms, and hyperoxia appears to worsen VILI. Antioxidants have been considered as a potential therapeutic strategy for preventing and treating ARDS, although clinical trials have so far been disappointing (see Therapy ).
Genetic Determinants
To date, relatively little is known about which genes might affect the development or prognosis of ARDS. Genome-wide association studies have suggested a number of candidate genes, including those coding for angiopoietin 2 and the angiotensin-converting enzyme, that might affect the incidence and/or outcome of ARDS. Subsequent studies have reported conflicting results. The heterogeneity of patients with ARDS is likely to make it difficult to identify clinically important genetic associations except in clearly defined subsets of patients.
Mortality and Complications
Mortality
The mortality rate for ARDS has decreased over the past 10 to 15 years, with a number of studies describing a decline in the rate from more than 60% to less than 40% since 1993. Mortality rates in adults and children with ARDS appear to be similar. The reasons for the improvement in survival over time are not known, although some have attributed it to better supportive care in the intensive care unit and the use of lung-protective strategies.
Predictors of Poor Prognosis
Despite the prominence of hypoxemia among the clinical manifestations of ARDS, early trials did not find that the severity of hypoxemia early in the course of the illness was a good predictor of subsequent mortality. Pulmonary injury scoring systems, such as the Lung Injury Score and the ARDS score, have been shown to predict a prolonged (>2 weeks) requirement for intubation and ventilation, while scoring systems that measure the overall severity of illness, such as the Simplified Acute Physiology Score, correlate better with survival. The classic teaching is that patients with ARDS do not usually die of refractory hypoxemia, which may seem paradoxical given that hypoxemia is frequently the focus of resuscitative efforts. In fact, most patients with fatal ARDS die of sepsis and multiorgan failure. The explanation for this apparent paradox is unknown, although it has been hypothesized that injurious mechanical ventilation during the course of ARDS may be involved. As discussed earlier, ventilation using excessive tidal volumes has been shown to cause elevations in pulmonary and systemic cytokine levels, and has been linked in an animal model to apoptosis of renal cells and renal dysfunction.
The mortality rate from ARDS varies depending on the precipitant. The highest risk of death has consistently been reported in sepsis, while ARDS in the setting of major trauma has a much better prognosis. In addition, it is known that chronic liver disease, older age, chronic alcoholism, and nonpulmonary organ dysfunction are associated with higher mortality from ARDS. Other predictors of death from ARDS have included a history of organ transplantation and infection with human immunodeficiency virus, while one study described a higher mortality rate in men and also in African-Americans compared to non–African-Americans.
As mentioned earlier, risk factors for ARDS have been classified as being pulmonary or nonpulmonary in origin, thereby leading to an injury to the lung by direct or indirect means. It remains unclear whether this distinction has prognostic importance. In one prospective cohort study of ARDS patients, investigators found a trend toward higher mortality in patients with a pulmonary precipitant, although the difference was not statistically significant. In contrast, the ARDS Network investigators retrospectively analyzed the data from their large randomized study of low tidal volume ventilation versus traditional tidal volume ventilation. While confirming that the mortality rate for ARDS was highest in patients with sepsis and lowest in patients with trauma, there was no difference in mortality, days off the mechanical ventilator, or the development of organ failure between patients with pulmonary or nonpulmonary risk factors. In addition, there was no statistically significant evidence that the low tidal volume strategy was less efficacious in any subgroup. A meta-analysis came to a similar conclusion. The consensus group that developed the Berlin definition of ARDS decided not to include pulmonary versus nonpulmonary ARDS as distinct categories.
Because hypoxemia is not a reliable predictor of mortality from ARDS, investigators have searched for other lung-specific markers of prognosis. In one prospective study of 179 patients with ARDS, a multiple logistic regression was performed to identify which clinical and physiologic variables predicted mortality. The analysis found that the dead space fraction (as calculated by the Bohr equation) was elevated in ARDS and was an independent predictor of mortality. For every increase of 0.05 in the dead space fraction, the odds of death from ARDS increased by 45%. The mechanism of this association is not known: it is possible that the pulmonary vascular injury in ARDS that accounts for the increase in dead space may be important to overall outcome.
The development of pulmonary fibrosis is also thought to connote a worse prognosis. Elevated procollagen III levels, thought to be indicative of collagen synthesis, have been found in the pulmonary edema fluid of patients with ARDS and have been shown to correlate with increased mortality. In another study, 22 of 25 consecutive patients with ARDS underwent transbronchial biopsies of the most abnormally appearing areas on the chest radiographs. In those whose biopsies showed any fibrosis, the mortality rate was significantly higher than in patients whose biopsies showed no fibrosis. Of note, there is evidence in animal models that the stress induced by mechanical ventilation may lead to lung fibrosis via epithelial-mesenchymal transition.
Finally, there is extensive interest in the identification of biomarkers to predict outcome from ARDS. For instance, associations have been reported between elevated levels of cytokines such as IL-6 and IL-8 and growth factors such as angiopoietin 2 and a poor prognosis from ARDS. However, even a combination of biomarkers when used together with clinical predictors is only slightly more predictive for mortality than the clinical predictors alone.
Complications
ARDS is complicated by ventilator-associated pneumonia in about 30% to 65% of cases (see also Chapter 34 ). In this setting, ventilator-associated pneumonia usually develops more than 5 to 7 days after the onset of mechanical ventilation and is often preceded by colonization of the lower respiratory tract by potential pathogens. The likely organisms include nonfermenting gram-negative rods, methicillin-resistant Staphylococcus aureus , and Enterobacteriaceae. Although the development of VAP prolongs the duration of mechanical ventilation in ARDS, it does not appear to increase mortality. Making a definitive diagnosis of VAP in patients with ARDS can be challenging, because patients with ARDS already have radiographic abnormalities, and not uncommonly have leukocytosis and fever. If diagnostic techniques such as bronchoalveolar lavage or protected specimen brushes are used, the yield is higher when the lung is sampled bilaterally and when the patient is off antibiotics.
Another feared complication of ARDS is barotrauma (pneumothorax, pneumomediastinum, subcutaneous emphysema, eFig. 100-1 ) due to the effect of positive pressure ventilation in heterogeneous lungs with diminished compliance. Because most patients with ARDS will be supine (rather than erect), diagnosing a pneumothorax requires vigilance; the radiographic appearance of a pneumothorax is different and can be subtler in the supine patient (e.g., air in the costophrenic angle, the “deep sulcus” sign). Data from a number of prospective studies suggest that the incidence of barotrauma in ARDS currently is about 10% or less.
Therapy
Supportive Care
One of the first goals of therapy in ARDS is to treat the underlying cause. In particular, patients with sepsis may respond to aggressive source control, including antibiotics and, when appropriate, surgical débridement and drainage. In patients with ARDS and sepsis of unknown origin, both the lung and the abdomen should be considered and excluded as foci of infection. Additional goals are the prevention of complications and the provision of supportive care (e.g., nutrition, ventilation) to allow the body time to heal. Typically, such treatment should include prophylaxis against gastrointestinal stress ulceration and deep venous thrombosis.
Hemodynamic Management
The optimal approach to hemodynamic management in ARDS has become less controversial with the publication of studies comparing different strategies. Before these studies, it had been unclear whether clinicians should attempt to diurese to decrease pulmonary edema at the expense of potentially causing hypovolemia and shock or to liberalize fluids to maintain perfusion.
This issue was addressed by a large multicenter randomized controlled trial from the ARDS Network, made up of investigators at multiple American hospitals. The trial randomized 1000 patients with ARDS to one of two highly protocolized fluid strategies: through the administration of fluids, diuretics, or vasoactive agents, the two protocols targeted either a higher or lower intravascular pressure (as measured by a pulmonary artery or central venous catheter) for 7 days. Patients randomized to the conservative fluid strategy (targeting a lower intravascular pressure) had significant improvements in oxygenation and more ventilator-free and intensive care unit–free days than the patients in the liberal strategy. Importantly, patients in the conservative arm did not have a higher incidence of dialysis or shock than those in the liberal arm; in addition, mortality rates in the two arms were similar. Thus, the results of this study suggest that conservative administration of fluids is safe and beneficial to patients with ARDS.
At first, this recommendation may seem at odds with the principle of early goal-directed therapy, whereby patients with sepsis are aggressively resuscitated with fluid in accordance with the randomized controlled trial reported by Rivers and coworkers. However, the apparently disparate results of these clinical trials are not difficult to reconcile. First, it is important to note that patients in the ARDSNet study were enrolled an average of 24 hours after meeting the criteria for ALI, much later than the 6-hour window in the study by Rivers. In addition, the study protocol of the ARDSNet trial was strictly designed to avoid aggravating or inciting shock or pulmonary edema.
One last caveat is that the ARDSNet study used a very complex algorithm for fluid administration that may not be widely adopted. A simplified protocol has since been proposed but has not been validated. In the meantime, we recommend that clinicians manage fluids conservatively in patients who are not in shock, nonetheless taking care to avoid overdiuresis and hypovolemia.
Clinicians had historically inserted a pulmonary artery catheter into patients with pulmonary edema to help establish the diagnosis and to guide therapy. The consensus from numerous clinical trials is that, for most patients, the information from a pulmonary artery catheter does not improve outcome. For this reason, we do not recommend the routine use of these catheters in patients with ARDS.
Which fluid to use in patients with ARDS remains an open question. The use of starch-based colloids has fallen out of favor in patients with severe sepsis, because of a randomized trial that found a higher incidence of renal failure in patients with severe sepsis receiving 10% pentastarch compared to patients receiving Ringer lactate. Whether pentastarch has the same detrimental effect in patients with ARDS without severe sepsis is unknown. On a theoretical basis, the use of albumin is appealing, because it would increase intravascular oncotic pressure and predispose to less pulmonary edema. A small placebo-controlled study in ARDS demonstrated that a regimen of albumin and furosemide infusions over 5 days caused a substantial and statistically significant improvement in oxygenation accompanied by a decrease in heart rate. However, most of the patients had ARDS as a result of trauma, with less than 5% having sepsis. There were no differences in important clinical outcomes (e.g., mortality), although the study was not powered to address these issues. A follow-up study established that the beneficial effect on oxygenation was due to the albumin, not the furosemide. Although a large clinical trial of patients admitted to the intensive care unit has concluded that albumin was as safe as crystalloid, the study did not specifically enroll patients with ARDS and only looked at short-term outcomes. It is also important to remember that as a blood product, the administration of albumin is associated with a very small but finite risk of transmissible diseases. Thus, pending further trials, the role of albumin in the management of patients with ARDS is unclear.
Nutrition
It has been hypothesized that manipulations in diet could enhance the immune system and improve the outcome of inflammatory diseases such as sepsis and ARDS. Such strategies have involved supplementing enteral feeds with one or more of arginine, glutamine, omega-3 fatty acids, and antioxidants.
One small randomized study examined the effect of a modified enteral feed containing eicosapentaenoic acid, gamma-linolenic acid, and various antioxidants compared to a control enteral feed in patients with ARDS. The authors found that the modified feed improved oxygenation, reduced the number of neutrophils in alveolar lavage fluid, decreased length of stay, and decreased the requirement for mechanical ventilation. In a more recent study, modified enteral feeds (containing eicosapentaenoic acid, gamma-linolenic acid, and various antioxidants) also improved oxygenation, although clinically important outcomes were unchanged. Many other studies of modified enteral feeds (often called immunonutrition) have been conducted in less well-defined populations of critically ill patients, with conflicting results. A meta-analysis on the topic highlighted the heterogeneity of the studies and suggested that the effect of immunonutrition varied depending on the group of patients being studied. At this point, the role of immunonutrition in the management of ARDS remains unclear.
A related issue in this area has been how much enteral nutrition should be given. In a recent randomized controlled trial, the rate of enteral feeding (e.g., at a regular rate versus a low, so-called trophic rate that delivered one third as many calories) did not affect the outcome of ALI.
Pharmacotherapy
Attempts to develop pharmacologic therapies for ARDS have been frustrating and largely unsuccessful, with no pharmacotherapies that unequivocally reduce mortality from ARDS, despite a multitude of randomized controlled trials of dozens of potential agents. Despite the heterogeneity of the agents that have been evaluated, three generalizations can be made:
- 1.
Despite showing effectiveness in vitro or in animal studies, most potential therapies have failed to reduce mortality or other important clinical outcomes in human clinical trials.
- 2.
A number of agents improve oxygenation but do not affect mortality from ARDS.
- 3.
Post hoc analyses of subsets of patients from a number of studies of various agents suggest benefit, but prospective data are lacking.
The following section reviews the biologic rationale for various potential therapies for ARDS, with an emphasis on evidence from clinical trials when available.
Corticosteroids.
Because of the presumed inflammatory pathophysiology underlying ARDS, a number of trials of high-dose corticosteroids have been performed. In some, the goal was the prevention of ARDS in patients at risk (e.g., with septic shock), while in others, steroids were given in established ARDS. The usual regimen was methylprednisolone 30 mg/kg every 6 hours for 1 to 2 days. None of the trials using this treatment regimen showed any benefit from the use of steroids, and one showed a higher incidence of infection in patients who received steroids. More recently, the use of steroids has been contemplated later in the course of ARDS, during the fibroproliferative phase. Persistently elevated plasma cytokine levels have been shown to correlate with worsened survival from ARDS, prompting some to theorize that late ARDS (>7 days after onset) is characterized by persistent inflammation that might be responsive to treatment with steroids. A small study randomized 24 patients with late ARDS to 2 mg/kg of methylprednisolone (followed by a 32-day taper) or placebo. Patients in the steroid group had lower mortality, improved oxygenation, decreased organ dysfunction, and earlier extubation, but also had a higher (but not statistically significant) rate of infection. However, these data are difficult to interpret because of the small sample size and the number of patients who crossed over to the alternate therapy.
A randomized placebo-controlled trial of 180 patients with ARDS of at least 7 days’ duration was subsequently performed by the ARDS Network. Patients were randomized to either placebo or a single dose of 2 mg/kg of methylprednisolone followed by 0.5 mg/kg every 6 hours for 14 days, then every 12 hours for 7 days, then tapering. Although the patients randomized to steroids showed improvements in the number of ventilator-free and shock-free days (during the first 28 days) as well as in oxygenation, there was no difference in 60-day mortality. A subgroup analysis revealed that patients who received steroids more than 14 days after the onset of ARDS had a significantly increased 60- and 180-day mortality rate. The issue of whether to use steroids in late ARDS remains controversial, but it seems reasonable to consider steroids in patients who are not improving between about days 7 and 14 in that benefit has been suggested in subgroups and harm has not been proven.
Vasodilators.
Prostaglandin E 1 is a vasodilator that has been studied as a potential therapy for ARDS, based largely on its putative anti-inflammatory properties. In vitro and preclinical studies in animals suggested that prostaglandin E 1 given parenterally, especially when administered in a liposome, had the potential to decrease neutrophil activation. Despite promising early data, a large randomized double-blind multicenter trial of liposomal prostaglandin E 1 found no improvement in survival or in ventilator dependence even though the drug improved oxygenation.
Prostacyclin is another vasodilator which, when administered by nebulizer, acts selectively on the pulmonary vasculature. Because the aerosolized solution tends to go to the better ventilated areas of the lung, vasodilatation of the branches of the pulmonary artery that supply these areas lead to improved matching and improved oxygenation. Although prostacyclin has been used as rescue therapy for refractory hypoxemia and is well tolerated, there are no large randomized studies and no placebo-controlled studies of its use in ARDS.
NO is a highly reactive gas formed endogenously by NOS from the amino acid arginine. It stimulates cellular guanylate cyclase, leading to increased cyclic guanosine monophosphate levels. It acts as a potent vasodilator, and when given by inhalation, NO causes vasodilatation of the pulmonary circulation. NO is rapidly inactivated in the bloodstream by combining with hemoglobin to form methemoglobin, which is usually rapidly metabolized and does not accumulate to levels that are thought to be toxic (i.e., methemoglobin < 5%). Because of this rapid inactivation, NO is a selective vasodilator that does not affect the systemic circulation. Like aerosolized prostacyclin, NO causes the most vasodilatation in the areas of the lung that are best ventilated, thereby improving matching. In addition, NO has both antiinflammatory and proinflammatory properties, although the contribution of these properties to its effects in a clinical setting is unclear. NO can also react with oxygen and water to form toxic metabolites, such as NO 2 and nitrous and nitric acid, although at concentrations of NO of less than 40 ppm, this problem is not usually clinically significant. A soda lime absorber can be placed in the inspiratory limb of the NO circuit in order to remove any NO 2 before the inspired gas reaches the patient.
In the largest randomized, double-blind, placebo-controlled study of NO in ARDS to date, more than 170 patients were randomized to different doses of NO (from 1.25 to 80 ppm) or placebo. Although approximately 60% of patients had a significant improvement in oxygenation within 4 hours of NO administration, there was no difference in survival or in liberation from mechanical ventilation between patients receiving NO and those in the placebo group. In addition, the initial improvement in oxygenation from NO was not sustained over the course of study. There were few adverse effects of NO, and for patients who were administered less than 40 ppm, methemoglobin and NO 2 levels were the same as in the placebo group. The results of this study confirmed the findings of other smaller unblinded trials of NO in ARDS and were repeated in a systematic review. In another large randomized but unblinded study of NO in ARDS, a higher proportion of patients in the NO group required renal replacement therapy than in the control group. A meta-analysis of trials using NO for ARDS concluded that, while NO caused initial improvement in oxygenation, it had no impact on survival and was associated with an increased risk of renal dysfunction. We suggest that NO should not be used routinely in the treatment of patients with ARDS, other than perhaps as a “rescue” therapy for extreme life-threatening hypoxemia. In fact, in a recent individual patient meta-analysis, inhaled NO did not improve mortality in patients with ARDS regardless of severity as assessed by arterial P o 2 /Fi o 2 ratios as low as 70.
Surfactant.
As discussed in the section on pathogenesis, a number of abnormalities of surfactant have been described in ARDS. These include an increase in relatively inactive forms, inactivation of surfactant by proteins that have leaked into the alveolar space, damage to type II epithelial cells (which produce surfactant), and destruction of surfactant constituents by the inflammatory process. These changes, along with the efficacy of surfactant supplementation in the neonatal respiratory distress syndrome, led to the hypothesis that surfactant supplementation might be beneficial in ARDS. Data from animal studies and small case series were promising. A small randomized controlled trial administered bovine surfactant through an endotracheal catheter in patients with ARDS and showed in one subgroup of patients an improvement in oxygenation and a trend to decreased mortality.
However, enthusiasm for surfactant was greatly dampened by the results of a large multicenter randomized, blinded, placebo-controlled trial. In this study, investigators administered an aerosolized synthetic (protein-free) surfactant or saline placebo continuously for up to 5 days to patients with new onset (<48 hours) of sepsis-induced ARDS. There was no physiologic or clinical benefit from the surfactant. Despite its methodologic rigor, the study has been criticized because less than 5% of the administered dose of surfactant was thought to have reached the distal lung. In addition, the lack of surfactant proteins in the synthetic surfactant may have diminished its ability to reduce surface tension. Because of these issues, the role of surfactant supplementation continues to be studied.
In a phase I/II trial, 40 patients with new-onset ARDS were randomized to a surfactant protein C-based preparation (given up to four times over 24 hours) or to no drug. The surfactant was administered through a catheter placed in the endotracheal tube. The drug had no effect on oxygenation or on ventilator-free days, which were the primary outcomes of the study. The authors reported a significant decrease in IL-6 levels in bronchoalveolar lavage fluid from patients who received the surfactant preparation, although it is unclear whether this was a prespecified end point.
Finally, two phase III trials of the recombinant surfactant protein C-based preparation have been completed. The studies demonstrated that the surfactant improved oxygenation, but had no impact on mortality or ventilator-free days. Only one trial, using calfactant (a natural surfactant, distinct from previous trials) in pediatric patients with ARDS, has shown any mortality benefit ; whether this is due to the unique surfactant used in this study or to differences between the patients enrolled in this trial and the negative trials (e.g., adult vs. pediatric) is unknown. Whether surfactant will prove to be useful in well-defined subgroups of patients with ARDS remains an open question.
Antioxidants and Anti-inflammatory Agents (Other Than Steroids).
Oxidative stress has long been postulated to be involved in the pathogenesis of ARDS. In fact, lung injury due to hyperoxia is a commonly used model to study ARDS in animals. Reactive oxygen species form as a by-product of activation of neutrophils and macrophages; in addition, the requirement by many patients with ARDS for a high inspired fraction of oxygen may predispose to oxidative stress. Decreased levels of glutathione, a major endogenous scavenger of reactive oxygen species, have been observed in the alveolar fluid of patients with ARDS. Small clinical trials with N-acetylcysteine and procysteine were promising. Unfortunately, despite this optimism, larger clinical trials of antioxidants in ARDS have been disappointing. A multi-center trial of the antioxidant procysteine in ARDS showed no beneficial effect of the drug.
Various agents with putative anti-inflammatory effects have been tested in ARDS. These include ketoconazole, lisofylline, and (in patients with sepsis at risk for ARDS) the nonsteroidal anti-inflammatory drug ibuprofen. In separate well-conducted, randomized, blinded, controlled trials, none of these agents demonstrated benefit in ARDS. A prospective randomized controlled trial evaluated the effect of recombinant platelet activating factor acetylhydrolase at preventing ARDS in patients with severe sepsis. This trial of more than 100 patients showed no decrease in the development of ARDS in patients who received the drug; however, the mortality rate was lower in patients receiving an intermediate dose of the study drug. Other trials of so-called anti-inflammatory therapies in sepsis have been disappointing.
Novel Therapies.
As discussed earlier in the section on Pathogenesis, alveolar fluid clearance can be augmented by catecholamines. A small trial randomized 40 patients with ARDS to intravenous salbutamol or placebo for 7 days. Patients receiving salbutamol had a decrease in extravascular lung water but a higher incidence of arrhythmias. A follow-up study was performed in almost 50 intensive care units in the United Kingdom and enrolled more than 300 patients with ARDS. However, the trial was halted earlier than planned because of an increase in mortality in the group receiving intravenous salbutamol. A similar-sized trial of aerosolized albuterol (given every 4 hours for up to 10 days) for ARDS found no benefit. Thus, routine use of β 2 -agonists cannot be recommended in ARDS.
Stem cell therapy for ARDS is being explored. Beneficial effects have been noted in animals with endotracheal and intravenous delivery of stem cells and may be augmented by using stem cells transfected with ANGPT1. A schematic of novel potential therapeutic approaches is shown in Figure 100-7 .
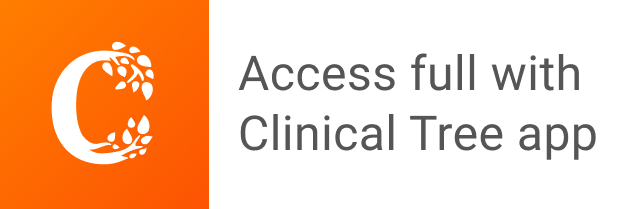