Definition
Shock may be defined as acute circulatory failure characterised by inadequate tissue perfusion and cellular damage. The inability of the circulatory system to deliver adequate quantities of oxygen to meet cellular demand is called cellular dysoxia. Therefore, the pathophysiological feature of shock is the imbalance between oxygen delivery (DO2) and cellular oxygen consumption (VO2).
Pathophysiology
It should be considered that although the mismatch between oxygen supply and demand is the main pathophysiological determinant of shock, the acute circulatory decompensation responsible for the altered perfusion of shock can be induced by four main mechanisms.
Circulatory insufficiency in shock can result from one or a combination of these mechanisms, leading to four main patterns of shock: hypovolaemic, cardiogenic, obstructive and distributive shock.
Hypovolaemic Shock
Acute loss of circulating volume (haemorrhage, loss of fluids) reduces venous return, which leads to severe hypovolaemia and consequent circulatory impairment.
Cardiogenic Shock
Acute cardiac failure, as occurs in acute myocardial infarction, myocardial ischaemia, cardiomyopathies and arrhythmias, is the main cause of cardiogenic shock. Dysfunction of heart pumping leads to low cardiac output (CO) and circulatory failure. Although the underlying mechanisms of hypovolaemic and cardiogenic shock are different, circulation is impaired, and oxygen delivery is decreased, with consequent tissue hypoperfusion.
Obstructive Shock
The main cause of circulatory failure is obstruction of circulation, as occurs in pulmonary embolism, pneumothorax and cardiac tamponade. Mechanical obstructions of ventricular ejection render the heart unable to pump adequate stroke volume (SV), resulting in low CO and tissue hypoperfusion.
Despite the different underlying pathophysiological mechanisms, in each of these three shock states (hypovolaemic, cardiogenic and obstructive), blood flow is significantly decreased, and oxygen delivery to peripheral tissues by the circulation is surpassed by cellular oxygen consumption.
Distributive Shock
Loss of vascular tone and consequent reductions in systemic vascular resistance (SVR) alter the distribution of blood flow, as in severe sepsis, major trauma and anaphylaxis. In this pattern of shock, the cause of circulatory failure is misdistribution of blood flow.
The macrocirculation is defined at a haemodynamic level in terms of CO and DO2, where, at stable concentrations of haemoglobin, CO is the main determinant of DO2.
The microcirculation regulates blood flow and cellular oxygen supply according to cellular metabolic requests. This regulation consists of increased tissue perfusion and increased cellular metabolism, which occur in response to long-term signals from cells under different metabolic conditions. Microcirculatory dysfunction is responsible for tissue damage and organ failure despite adequate systemic blood flow and optimisation of the macrocirculation.
In septic shock, the microcirculation is profoundly affected, and tissue perfusion is consequently altered. Vasoplegia and vascular hyporeactivity are the most important features of the haemodynamic impairment of septic shock. The loss of control of vascular tone seems to be related to an imbalance between vasodilators and vasoconstrictors and is associated with high mortality rates. The loss of vascular tone is induced by inflammatory mediators and endogenous vasodilatory mediators produced by cells under oxidative stress conditions and increased metabolic activity. Markers of both systemic inflammation and cellular oxidative metabolism (pCO2, lactate, K+, H+, nitric oxide (NO), prostaglandins, interleukin-1, adenosine, inorganic phosphate) can be commonly detected in patients with altered cardiovascular function and shock. The actions of these products result in endothelial dysfunction and altered microvascular perfusion. The altered modulation between vasodilating and vasocostricting agents is responsible for vasoplegia and for hyporeactivity of arterial vessels to vasoconstriction, which is common in septic shock patients. The predictive value of these mediators as biomarkers is lower than that of the concentration of plasma lactate; therefore, their routine use as biomarkers is not recommended.
Ventriculoarterial (VA) Coupling
VA coupling reflects the interaction between cardiac function and the vascular system. When the two components of the cardiovascular system, the heart and the arterial tree, are coupled, maximal efficiency is achieved. VA coupling can be defined as the ratio of arterial elastance (Ea) to ventricular elastance (Ees) (Ea/Ees), as proposed by Suga in 1969. When the heart pumps a quantity of blood that matches the capability of the arteries to receive it, the system is optimised. Under different physiological and pathological conditions, the heart provides adequate flow and pressure to ensure tissue perfusion. The arterial system adapts to match the continuous variations in blood flow. VA decoupling occurs in most acute altered haemodynamic states. This means that cardiovascular function is impaired and that global perfusion is suboptimal. Both increased and decreased arterial tone negatively affect VA coupling in shock. Loss of vascular tone due to septic shock and vasoconstriction imposed by either autoregulatory mechanisms induced by hypotension or the use of vasopressors are the main causes of changes in Ea. Ees can be decreased by alterations in LV contractility, which occurs in both cardiogenic shock and septic shock when myocardial depression occurs.
Epidemiology
All types of shock can occur in critically ill patients admitted to the cardiothoracic intensive care unit (CT ICU), and two or more patterns of shock may be present in the same patient. It is not uncommon for cardiogenic shock to be complicated by septic shock, and cardiogenic shock can complicate pre-existing septic shock. It should be considered that haemodynamic instability is one of the most common causes of admission to the ICU.
Diagnosis
Circulatory shock is commonly associated with haemodynamic instability characterised by moderate to severe arterial hypotension and signs of tissue hypoperfusion. Arterial hypotension, which is defined as a systolic blood pressure less than 90mmHg, a mean arterial pressure (MAP) <65mmHg, or a decrease ≥40 mmHg from baseline, is commonly present in patients with shock. However, hypotension is not required to diagnose shock, as recently emphasised by the revision of the recommendations of the 2006 guidelines for the management of patients with shock. Signs of peripheral hypoperfusion are more suggestive in the diagnostic evaluation of shock, along with increased plasma levels of lactate and decreased central oxygen saturation. The three sites that can be easily evaluated and most commonly reveal tissue hypoperfusion are the skin, kidneys and brain (Table 29.1). Patients with cardiovascular impairment generally present with non-specific symptoms, such as moderate to severe arterial hypotension associated with clinical signs of tissue hypoperfusion, such as increased concentrations of blood lactate, decreased central venous oxygen saturation (ScvO2), reduced urine output, cold skin and neurological symptoms, such as mental confusion or agitation. As recently reported in the literature, hyperlactataemia is so strongly associated with shock that it is assumed to be part of the definition of shock. Increased blood concentrations of lactate above the cut-off of 2 mEq/l represent severe metabolic alterations due to inadequate utilisation of oxygen carried to cells via the circulation (DO2/VO2 mismatch). Although lactate levels can also increase with normal tissue perfusion and adequate oxygenation, in most cases, especially in cases of acute circulatory failure, hyperlactataemia is associated with cellular dysfunction, and it negatively affects the outcomes of patients with all types of shock. Recently, lactate-guided therapy was proposed for patients with shock. Some authors have reported that this reduces hospital mortality by 20% every 2 hours for the first 8 hours of therapy. Plasma lactate levels should be routinely evaluated in patients exhibiting acute haemodynamic changes to assess peripheral organ perfusion; lactate values can also be helpful in determining the effectiveness of therapeutic strategies as normalisation of plasma lactate concentrations is correlated with improvements in tissue perfusion.
Table 29.1 Signs of systemic hypoperfusion at three levels
Skin | Inspection |
|
Kidney | Urine output | ≤0.5 ml/kg/hr |
Brain | Mental status |
|
ScvO2 may reflect DO2/VO2 mismatches in acute circulatory failure. Low ScvO2 (<70%) is assumed to reflect inadequate oxygen delivery to peripheral tissues. Nevertheless, normal or even high values of ScvO2 do not seem to be correlated with adequate oxygen transport.
Another indicator of adequate blood flow and tissue perfusion is the venoarterial carbon dioxide difference (pCO2 gap), the difference between central or mixed venous carbon dioxide partial pressure (pCO2) and arterial pCO2. A value ≥6 mmHg indicates low peripheral perfusion despite normal ScvO2 levels.
It should be considered that some patients have normal systolic blood pressure despite evident cardiovascular decompensation because of activation of compensatory mechanisms of autoregulation. Arterial vasoconstriction is the primary mechanism of immediate compensation in cases of circulatory failure. Increased SVR induced by arterial vasoconstriction allows systemic blood pressure to be maintained within a normal range, even in cases of large volume loss or severe cardiac dysfunction. This autoregulation mechanism also counteracts the loss of vascular tone, which is present in maldistributive shock, ensuring normal arterial blood pressure in the early phase of cardiovascular decompensation.
Autoregulation mechanisms ensure adequate blood flow and DO2 to tissues and organs at different systemic blood pressure levels; when blood pressure falls below the limits of autoregulation, haemodynamic instability manifests, and cellular injury occurs. Ultimately, the mismatch between DO2 and VO2 results from an imbalance between the macrocirculation and microcirculation.
Patients with cardiogenic shock should be further investigated with respect to cardiac function, and the use of inotropes is recommended to improve contractility and SV in severe heart dysfunction.
The use of vasopressors is indicated in cases of insufficient responsiveness to fluid administration.
Monitoring Cardiac Function and Haemodynamics in Patients with Shock
Cardiac function should be accurately evaluated in cases of acute circulatory dysfunction to define the type of shock, first distinguishing among cardiogenic, hypovolaemic and obstructive shock, which are all characterised by low CO. In addition, in patients with suspected septic shock, investigations of cardiac performance may provide information about global haemodynamic impairment and the eventual myocardial depression that frequently complicates septic shock.
Advanced haemodynamic monitoring may be required in patients with severe cardiovascular instability either to categorise shock or to evaluate responsiveness to therapy.
In this context, echocardiography has been recognised as the most useful tool to either evaluate cardiac function or allow haemodynamic assessments in patients with shock. Echocardiography is a non-invasive and reliable bedside method of detecting cardiac function, CO and preload in acute circulatory decompensation or in follow-up of patients with shock. Assessment of cardiac function is also recommended to determine either therapeutic strategies or the need for adjunctive haemodynamic monitoring. The main limitation of echocardiography is that it cannot be continuously performed; therefore, haemodynamic monitoring should be performed in critical patients for whom continuous information about haemodynamics is required.
Fluid Responsiveness
For these reasons, therapeutic interventions to restore adequate circulation and global perfusion should be based on preload, afterload and cardiac function. Although fluid resuscitation is the first line therapy in patients with shock, the risks associated with unnecessary fluid administration have recently been demonstrated. In this context, in cases of acute circulatory failure, appropriate fluid administration and the response of the patient to an increased preload should be adequately investigated. The role of fluid responsiveness (FR) as a guide for fluid resuscitation in shock has been progressively emphasised. FR represents the ability of the left ventricle to increase SV after administration of fluids. An increase of 15% in SV or CO after administration of a bolus of fluid indicates the so-called fluid response. FR can be evaluated either by static or by dynamic methods, as reported in Table 29.2. Although central venous pressure (CVP) and pulmonary artery occlusion pressure (PAOP) are commonly employed to assess left ventricle (LV) and right ventricle (RV) preload, respectively, their prognostic value is poor. Measurements of cardiac chamber area and volumes detected by echocardiographic assessments and the thermodilution method are good indicators of preload but have poor prognostic value. The static diameter of the inferior vena cava (IVC) has been proposed as an index of volaemia because it is reduced in hypovolaemic patients. Its use is not recommended in the detection of FR. While static measures indicate preload with more or less accuracy, depending on the method employed, dynamic measures evaluate the ability of the LV to increase preload after fluid administration. Dynamic measures are based on the physiological interaction between the heart and lungs in patients under positive pressure mechanical ventilation (MV). The effects of positive pressure ventilation on RV ventricle preload and afterload have been well demonstrated. During the respiratory cycle, RV preload decreases (reduction in the venous return), and RV afterload increases because of an increase in transpulmonary pressure. The decrease in RV preload negatively affects LV filling and LV SV. The changes induced on LV SV by positive pressure ventilation and arterial pulse pressure can be used to detect FR.
Table 29.2 Static and dynamic methods for the evaluation of fluid responsiveness
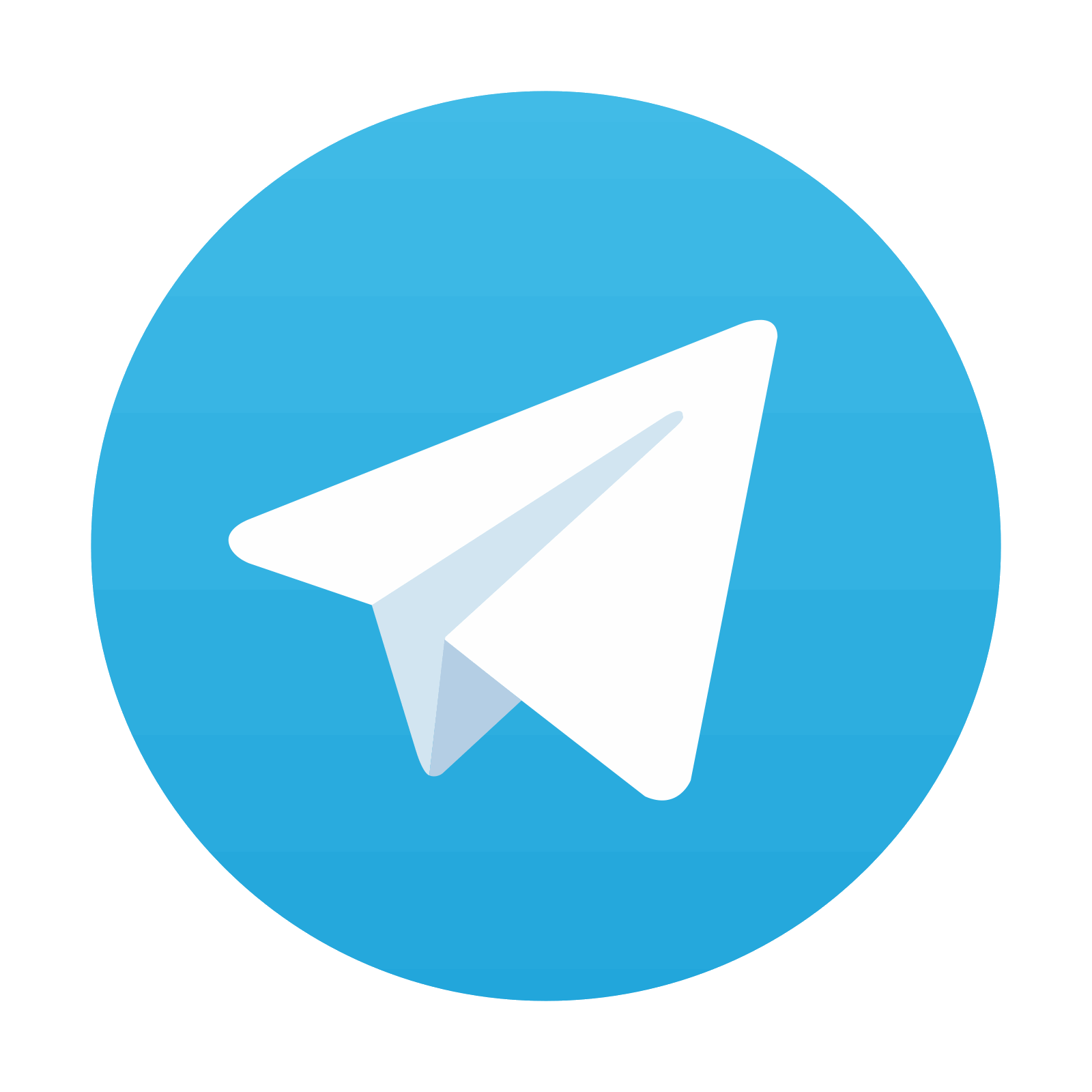
Stay updated, free articles. Join our Telegram channel
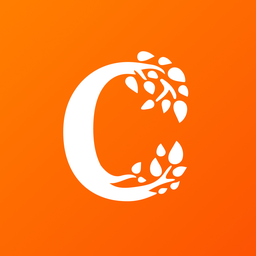
Full access? Get Clinical Tree
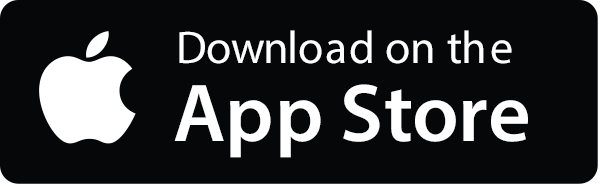
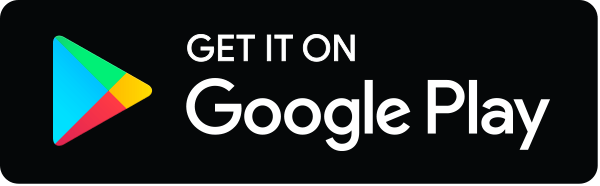
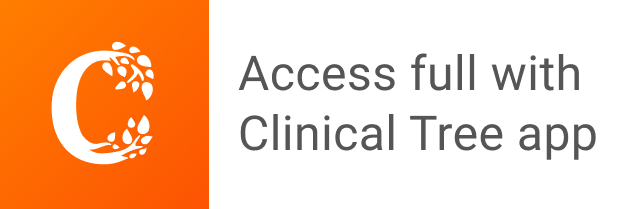