Chapter 3
Vessel Wall Biology
Bauer Sumpio, Jason Chin
The normal functioning of complex multicellular organisms relies on the integrity of elaborate fluid transport networks in both the cardiovascular and lymphatic systems for the delivery of nutrients to tissues and the removal of waste products. These systems perform crucial metabolic and mechanical functions and are extremely responsive to various physiologic and pathologic conditions.1 The arterial network reacts to and often controls hemodynamic forces both acute and chronic through the constriction or dilatation of vessel size and also via activation of various signaling pathways. Its venous counterpart likewise is an adaptable system with its own unique mechanisms for accommodating the various hemodynamic stresses of the body. The lymphatic system is a network of vessels distinct from but closely related to the cardiovascular system that augments its fluid transport and immunologic functions. This chapter discusses vessel wall biology, including wall anatomy and key functional implications as they relate to the normal physiology of the cardiovascular and lymphatic systems (Table 3-1).
Arterial Wall Anatomy
The arterial wall exhibits a common organizational structure and composition throughout the vascular system, although proportions of components may fluctuate through different regions of circulation. The arterial wall consists of organized connective tissue composed of cells and matrix fibers that are arranged in three tunicae: intima, media, and adventitia (Fig. 3-1).
Figure 3-1 Anatomy of the vessel wall. Arteries and veins maintain the same basic structure, which consists of the tunica adventitia, tunica media, and tunica intima, although capillaries consist of no more than endothelium, connective tissue, and support cells called pericytes. (Redrawn from Hast J: Self-mixing interferometry and its applications in noninvasive pulse detection [dissertation]. Finland, 2003, University of Oulu.)
Intima
The intima is the innermost luminal layer of the arterial wall, extending from the lumen to the internal elastic lamina. The intimal luminal surface is lined by the endothelium. The actual intimal layer is very narrow consisting primarily of a few scattered leukocytes, smooth muscle cells (SMCs), and connective tissue fibers. The matrix fibers consist mainly of elastic and collagen fibers, and proteoglycans.
Endothelium
The vascular endothelium is a continuous layer of flat polygonal endothelial cells (ECs) that come into direct contact with blood flow. The endothelium participates in both physiologic and pathologic processes and reacts to physical forces, chemical signaling, and immunologic mediators.2 The endothelium is able to interact with its cellular and acellular environments and is an important regulator of vasomotor tone, hemostatic balance, permeability, cell proliferation, survival, and immunity.3
The endothelium provides a continuous lining throughout the arteries, capillaries, veins, heart valves, and endocardial surfaces. Cell thickness varies from less than 0.1 µm in the capillaries and veins to 1 µm in the aorta.3 The luminal surface of the endothelial cell is smooth and regular.4 The cells are covered by a glycoprotein coat, a glycocalix that is responsible for the antithrombogenic properties of the endothelial surface.5 The glycocalix reduces friction from blood flow and serves as a barrier for fluid loss through the vessel wall. Its thickness varies across the vascular tree.6 During inflammation, the glycocalix is sheared off, permitting the attachment of leukocytes and the transport of water from microvessels, and possibly initiating the development of atherosclerotic lesions.7
ECs, which are typically aligned in the direction of blood flow, tend to overlap immediately adjacent cells. The nature of the cell bonding is relevant to both the mechanical strength and the permeability of the endothelial layer. Membranes of adjacent cells are mainly parallel and separated by an intercellular space of 15 to 20 nm in size.5 Sites of firmer attachment include tight junctions (zona occludens) and adherens junctions (zona adherens). Tight junctions form a barrier to transport between ECs and help maintain polarity between the luminal and abluminal sides of the cells. At these sites, opposing cell membranes form a continuous band around the side of the cells, forming a zona occludens. This tight seal prevents the passage of molecules between the lumen and cell base. Large artery ECs have a well-developed system of tight junctions because of their exposure to high rates of pulsatile blood flow. Junctions tend to be tighter in the arterioles than in the capillaries and are quite loose in the venules. The postcapillary venules most likely possess a less dense system of tight junctions in order to mediate the inflammation-induced extravasation of leukocytes and plasma constituents. In contrast, the blood-brain barrier, protecting neural tissue from fluctuations in blood composition, has particularly rigid tight junctions.3 Adherens junctions are less common and exist primarily in large arteries. Their primary role is to permit inter–endothelial cell communication via movement of ions, metabolites, and regulatory factors.5
The ECs contain pinocytic vesicles, which regulate the permeability of the endothelial layer. The pinocytic vesicles are responsible for the movement of material from the lumen of the vessel into its wall. The vesicles exist primarily in muscular small blood vessels and are found in large numbers in the heart and lung and to a lesser extent in the retina and the brain.8
The endothelium may be continuous or discontinuous. A continuous endothelium may be fenestrated or nonfenestrated. Nonfenestrated continuous endothelium exists in arteries, veins, and capillaries of the brain, skin, heart, and lung. Fenestrated endothelium occurs in locations of increased filtration or transendothelial transport, including the capillaries of exocrine and endocrine glands, gastric and intestinal mucosa, choroid plexus, glomeruli, and renal tubules. Fenestrae are transcellular pores approximately 70 nm in diameter that extend through the entire endothelial layer.3 The majority of fenestrae have a 5- to 6-nm nonmembranous diaphragm across their openings, although fenestral density varies in differing vascular beds.9,10
Discontinuous endothelium exists in the liver and possesses larger fenestrations, ranging from 100 to 200 nm in diameter. These fenestrations lack a diaphragm and contain gaps or pores within individual cells.11
Basal Lamina
The basal lamina, which borders the ECs on their abluminal surfaces, forms a more or less continuous boundary between the endothelium and the immediately underlying intimal structure. It provides support for the regeneration and attachment of the endothelial layer, plays a role in blood vessel permeability, is involved in the initiation of blood clotting, and provides a barrier to cellular migration.12 As a specialized section of the extracellular matrix (ECM), it connects the EC compartment to subjacent cell layers and SMCs.13
The basal lamina consists of two zones, a clear inner zone (lamina rara) and a dense fibrillar zone (lamina densa), which lies next to the interstitial connective tissue.2 The clearer inner layer contains the glycoprotein laminin, whereas the dense layer is composed of type IV collagen. The basal lamina provides a pliable extensive bond, which permits ECs to comply with changes in configuration related to cardiac pulsation and to vessel torsion and flexion.5,14 Furthermore, discrete points of increased electron density and attachment have been observed between basal peripheral cytoplasmic dense bodies of ECs and the internal elastic lamina. These adhesion points most likely prevent slippage, buckling, and excessive overriding of the lining cells when shear stress increases.14
Mechanically, the basal lamina aids in strengthening the vascular wall. Collagen type IV chains form a covalently stabilized polygonal framework and provide mechanical resistance. Laminin self-assembles to form a second polymer network and connects the ECs to the collagen.13,15 Entactin binds laminin and collagen. Fibronectin transmits mechanical forces between integrin receptors on vascular SMCs and the extracellular matrix in order to redistribute the mechanical load within the arterial wall.16
In normal functioning, the monolayer of endothelium is in a quiescent state, in which cell-cell contact inhibits proliferation, resulting in a low mitotic index and contributing to the stability and integrity of the vessel wall.2 In this state, the ECs form complex cell-to-cell junctions, synthesize and secrete ECM components, including fibronectins, and secrete a basement membrane composed of laminin, proteoglycans, and collagen.
The basal lamina is formed by glycoproteins; adhesion molecules, such as laminin, fibronectins, entactin, and thrombospondin; various proteoglycans, such as heparan sulfate chains; and microfibrils of collagen types IV and V approximately 5 nm in diameter.17 Endothelium is responsible for the secretion and deposition of collagen IV to form the basal lamina.12
The underlying reticular layer of the endothelium is composed of collagen types I and III, which are produced by both ECs and SMCs. Evidence suggests that the ECM components influence cell phenotype in ECs and SMCs. In culture, endothelial cell phenotype is influenced by growth media, surface, and the age of the cultures. Further, culture of these cells on various surfaces, including collagens, fibronectins, and reconstructed basement membranes, demonstrates different rates of production of gap junctions, actin, messenger RNA (mRNA), and protein, cell proliferation, and membrane specialization.18–20
Proteoglycans.
Proteoglycans are macromolecules that can influence viscoelasticity, permeability, lipid metabolism, hemostasis, and thrombosis. Endothelial cells in culture produce mainly heparan sulfate–like proteoglycans and smaller amounts of dermatan and chondroitin sulfate. The heparin sequences are chemotactic for ECs and are able to modulate endothelial cell growth factor to increase its mitogenic capacity.
Collagen.
Collagen is crucial to endothelial cell attachment to the subendothelial matrix to preserve the integrity of the monolayer. Type IV collagen is characteristic of the basement membranes and is synthesized by ECs and SMCs. Type IV has a high-proline triple-helix structure, which imparts flexibility to the binding sites of cells to the matrix and enhances endothelial cell adhesion and migration.21,22 Type V collagen is a pericellular collagen also produced by both ECs and SMCs. Its function is to bind interstitial collagen to cells or basal laminae. Of all interstitial collagen, types I and III are the most predominant. Type I is the major collagen in human aorta intima-media, and type III predominates in the subendothelium of younger individuals.
Laminin.
Laminin is a noncollagenous basement membrane that plays a pivotal role in mediating cell–basement membrane interactions and accelerating the attachment of ECs and heparan sulfate to type IV collagen.
Fibronectin.
A large glycoprotein composed of two disulfide-linked subunits, fibronectin is able to promote the adhesion of molecules, the spread of mesenchymal and epithelial cells, and the proliferation and migration of embryonic and tumor cells. It also regulates cell differentiation, shape, and cytoskeletal organization. Microvascular and human umbilical vein ECs preferentially attach and migrate to fibronectin rather than laminin or collagen.2
Internal Elastic Lamina
The internal elastic lamina (IEL) is a layer of elastic fibers 70 to 100 nm in thickness. At normal intraluminal pressure, the IEL straightens to form a circular band on transverse sections of the intima. Experimental evidence suggests that both abluminal SMCs and luminal ECs are capable of elastin formation; they are collaboratively responsible for IEL synthesis.23,24 The IEL separates the subendothelial intimal layer from the medial layer. It is marked by collagen- and proteoglycan-based fenestrations, which permit flow, driven by a pressure gradient, across the arterial wall tissues from interendothelial clefts into the intimal layer.5,25,26
Elastin is organized into fenestrated cylindrical lamellae that are separated from neighboring lamellae by single layers of SMCs.27 Lamellar units are determined prenatally, and postnatal changes in thickness or circumference of the media accompany parallel changes of the thickness and circumference of the elastic lamellae.28,29
It has been suggested that in large arteries, the internal elastic lamella and elastic lamellae function as barriers to macromolecular accumulation in the vascular wall. Structural defects within the IEL have been directly implicated in the onset of intimal thickening in human arteries.25 Fragmentation of the elastic laminae and disruption of medial layers are common characteristics of advanced plaques in apolipoprotein E knockout mice and have also been found in earlier stages of plaque development.30 It is believed that a defective IEL results in abnormal attachment with ECs, causing gaps in the endothelial surface and thereby facilitating the entry of macromolecules, lipids, and leukocytes into the intima.25,31,32
Although macromolecules are believed to cross the normal arterial endothelial cell layer through vesicular transport, macromolecules are believed to cross the elastic lamina via diffusion through the fenestrations. The diffusion rate is inversely dependent on its size. Therefore, as the size of the macromolecule increases, the resistance of the endothelium does not change but IEL resistance strengthens.33 Owing to this difference in transfer rate, the IEL most likely plays a significant role in regulating macromolecular accumulation in the intima, in restricting plasma-borne macromolecules from entering the media, and in regulating discrete microenvironments between the intima and the media.33
Media
The tunica media extends from the internal elastic lamina to the adventitia. The medial layer is a porous heterogeneous medium consisting of an ECM phase with embedded SMCs. Under normal conditions, SMCs in the tissue matrix beneath the IEL are shielded from the direct shear forces of flowing blood. At low and physiologic pressures, the media is the chief determinant of arterial wall properties. Its composition and microarchitecture are designed to ensure arterial stability. Arterial media elasticity dictates arterial distensibility and the capacitive effects of larger arteries.34 Its elasticity also affects the mechanical load-bearing function of the arterial wall. The metabolic state of the media also plays an important role in the pathogenesis of atherosclerotic lesions.
The media consists of vascular SMCs, elastin, and collagen fibers all arranged in a highly organized fashion.35 Closely packed layers of SMCs in close association with elastin and collagen fibers distinguish the outer layer of the media in all intact arteries. The SMCs are surrounded by a basement membrane composed mainly of laminin, collagen IV, heparan sulfate proteoglycan, entactin/nidogen, and fibronectins, which prevent the proliferation and migration of the SMCs. This arrangement maintains the SMCs in a contractile state as opposed to the synthetic state.36 During the contractile state in normal arterial vessels, SMCs are differentiated and contain abundant contractile and intermediate filament proteins. Under certain physiologic and pathologic conditions, the SMCs abandon their contractile phenotype for an immature synthetic phenotype. This change permits their migration into the intima, proliferation, and secretion of ECM components.37
The SMC layers consist of groups of similarly oriented cells, each surrounded by a common basal lamina in a closely associated interlacing basketwork of type III collagen fibrils. The fibrils are arranged to tighten around the cell groups as the media is brought under tension. This configuration holds the groups of cells together and prevents excessive stretching or slippage.1 This highly integrated system implies that one element cannot be extended without the extension of the other. Elastin and SMCs work in tandem. It appears that at low and physiologic pressures, collagen fibers are slack and not substantially load-bearing.35 The thickness and abundance of collagen bundles do depend largely on vessel diameter. The average tangential tension per medial layer is approximately 2000 dynes/cm, regardless of species.38
Arteries are classified as either elastic or muscular according to the proportion of cellular and fibrous components.39 In elastic arteries, well-defined elastic lamellae and collagen bundles are prominent in the media whereas connective tissue fibers are less frequent. The prominent elastic arteries are those with large diameters and in close proximity to the heart—the aorta, the brachiocephalic trunk, and the iliac arteries. Elastic arteries consist of lamellar units, which are close associations of elastin, collagen, and SMCs. Between the lamellae, SMCs, collagen fibers, elastin microfibrils, and glycosaminoglycans are organized into functional units. Although the number of lamellar units depends on the arterial diameter, humans have from 40 to 60 units in the aorta, which are located approximately 12 to 17 µm in distance. from the lumen of the artery. The number of lamellar units decreases from the heart to the peripheral arteries.5
Muscular arteries predominate in the second- or third-order branches of the elastic arteries. The media consists primarily of SMCs and has fewer connective tissue fibers. Nonparallel branching elastin strands increase the capacity to change diameter under the influence of neurohumoral stimulation.
Electron microscopy has illuminated a further structural breakdown of arterial microarchitecture in elastic and muscular arteries. The media is composed of groups of fascicles, which are jointly oriented, elongated SMCs within an encompassing network of branching elastic fibers.35 Bundles of slack collagen fibers are interposed between the adjacent elastic fiber systems. Each fascicle is invested by a sheath of basal lamina and collagen fibrils, which are oriented in the same direction as the fascicle cells.5 Each fascicle is oriented in the direction of imposed tensile stress. The orientation of its elastin fibers affects the stress distribution throughout the wall.34 (In straight segments of aortas and arteries, fascicles are uniform in size and oriented primarily circumferentially. At bends and branching points, fascicles are smaller and less uniform in size and orientation.38
Adventitia
The adventitia extends from the external elastic lamina to a boundary that is rather difficult to define; it is usually contiguous with the perivascular connective tissue. The adventitia varies in thickness and organization. Whereas the aorta has minimal adventitial fibrous connective tissue, large muscular arteries contain prominent adventitial elastic and collagen fibers. Here, adventitia may be more prominent than media. Generally, adventitial cells are sparse and consist mainly of fibroblasts.
The adventitia contains vasa vasorum and nerves, which provide nutrition to the adventitia and media and contribute to the regulation of the medial smooth muscle function. The vasomotor nerve fibers induce vasoconstriction via adrenergic receptors or vasodilatation through activation of β receptors. Nervous stimuli are transmitted to the outer SMCs through neuromuscular junctions. The signal is transmitted to the inner cells by electrical coupling between adjacent SMCs.
Vasa vasorum are present in arteries with diameters larger than 200 µm. Also, when the medial layer has more than 29 lamellar units, the outer part of the media is irrigated by the vasa vasorum. Thus, nutrients are also provided from the luminal blood flow for the inner part of the media, and from the vasa vasorum for the outer layers of the media. The vasa vasorum are composed of small arteries, arterioles, capillaries, and venous channels, as well as nerves that mediate smooth muscle tone and contraction. Vasa vasorum arise from the parent artery at branch junctions and arborize in the adventitia. In thick-walled arteries, mural stresses and deformations may affect the vasa vasorum. Blood flow and hypertension may impair vasa flow. A lymphatic network in the adventitia collects the proteins, ions, and water from the blood and transports these substances through the vessel wall.
Arterial Wall System
Large Arteries
The arterial system commences with the aorta and larger arteries the primary functions of which are to provide a conduit for blood flow to the peripheral tissues and to smooth out the pulsations of intermittent ventricular ejection. The large arteries cushion the pulsations that occur during ventricular ejection. This cushioning function is characterized by the relationships between oscillatory pressure, flow, and frequency, which in turn depend on arterial diameter and elasticity.5
The aorta is the main trunk of the vascular system, originating at the upper section of the left ventricle. It is about 3 cm in diameter. The aorta arches backwards over the root of the left lung and descends within the thorax on the left side of the vertebral column. It then flows into the abdominal cavity through the aortic hiatus in the diaphragm and ends, significantly diminished in size, opposite the lower border of the fourth lumbar vertebra. At this 1.75-cm size, the aorta divides into the right and left common iliac arteries, marking the beginning of the large arterial system.
Medial cells in aortic sections are organized into groups of overlapping elongated cells, bracketed by elastic fibers. Successive layers of cells and their surrounding elastic fibers are separated from one another by a narrower, intervening acellular zone containing thick, crimped bundles of collagen fibers.
Because of their significant size, the aorta and the large arteries offer little resistance to blood flow. Large arteries store a large portion of the blood volume during systole and drain it during diastole. They are able to accommodate the stroke volume ejected with each contraction of the heart. This ability allows for propagation of the pulse and also offers dynamic resistance to the oscillatory components of pulsatile flow.
Blood pressure is the highest at the origin of systemic circulation, although decreases in blood pressure are not linear with vessel diameter or distance from the vascular tree.5 Blood pressure decreases to 30% to 40% of aortic pressure in vessels 250 to 50 µm in diameter. Significant pressure drop occurs in the terminal arterioles, which branch into small capillaries and possess diameters less than 100 µm (Fig. 3-2). In vessels smaller than 60 µm, no correlation has been found between central arterial pressure and microvascular pressure, suggesting that perfusion pressure is being controlled in these blood vessels and those with smaller diameters.40
Figure 3-2 Pressure drop through hamster cheek pouch circulation. MAP, Mean arterial pressure, measured in the femoral artery; VP, venous pressure, measured near external maxillary vein. Bars indicate standard error. (Redrawn from Mulvany M, et al: Structure and function of small arteries. Physiol Rev 70:922-949, 1990.)
The larger arteries appear to be predominantly under the control of regional resistance vessels, and there is little evidence that vasomotor changes in large arteries occur independently of those in small resistive arteries.41 Changes in caliber in large conduit arteries occur passively through local or systemic changes in transmural pressure and actively through changes in vascular smooth muscle contraction. Arteriolar vasomotion may induce systemic or local blood pressure changes that alter the diameter of the artery and its viscoelastic properties. Arteriolar constriction or dilatation may also modify arterial smooth muscle tone through the endothelium-dependent mechanism of high-flow dilatation. Evidence suggests that the tone of large arteries may be regulated actively. Studies in isolated vessels, animals, and humans suggest that large arteries respond directly to both autonomic reflex and neurohumoral stimulation.42
Chemical stimulation may exert a significant influence in the control of arterial tone. There are significant differences in the response to adrenoreceptor stimulation and antagonism between large and small arteries. The aorta is poorly innervated in comparison with peripheral arteries. However, in conjunction with highly sensitive vascular SMCs and an excess of α1-adrenoreceptors, larger arteries are sensitive to adrenergic stimulation.43 Sympathetic control of the vascular tone of large arteries is more sensitive to α-adrenergic blockade than that of smaller vessels.44
Small Arteries
Small arteries are the principal regulators of peripheral resistance with the ability to change diameter markedly in response to stimuli. Once in the peripheral regions, the diameter of individual arteries decreases while the percentage of total blood volume increases and leads to a fall in blood pressure. The amount of elastic fibers in the tunica media decreases owing to these physiologic changes in addition to having the lowest proportion of medial fibrous connective tissue. Initially it was believed that resistance arteries consist solely of arterioles with only one layer of SMCs and diameters of less than 30 to 50 µm. However, it has been shown that approximately 50% of precapillary resistance lies proximal to the arterioles and to vessels with diameters of 100 µm.45
Smaller arteries are considered muscular arteries. The tunica intima is thinner than in elastic arteries. The subendothelial connective tissue, aside from the internal elastic lamina, is difficult to discern. The tunica media demonstrates numerous concentric layers of SMCs and a few elastic fibers and collagen fibers. The external elastic lamina is clearly marked though incomplete in various areas. The thickness of the adventitia in the arterial wall is variable, being small in cerebral small arteries and taking up approximately half of the wall in other regions. Nerves remain contained within the adventitia and do not penetrate the media.46 The nerves, which are primarily adrenergic, consist of unmyelinated axons along which are interspersed varicosities. Axons remote from the adventitia-media border exist in bundles of up to 50. Closer to SMCs, axon bundles contain 1 to 11 axons. Although there are multiple junctions between axons and SMCs, the neuromuscular junctions in small arteries are less developed and specialized than in skeletal muscle.45
The tunica media in the small arteries contains a well-defined IEL with an undefined and almost nonexistent external elastic lamina.47 The number of SMC layers within the media of small arteries decreases relative to vessel diameter from approximately six layers in 300-µm vessels to a monolayer in 30- to 50-µm arterioles. The volume fraction of SMCs in the media of small arteries as seen by electron micrographs is from 70% to 85%, a fraction that is greater than in larger vessels.45
Within the tunica intima, the ECs form a normal continuous layer. The ECs of small arteries often project through fenestrations in the IEL and may come into contact with vascular SMCs in the tunica media.47 The subendothelial space of the tunica intima contains elastic fibers and collagen fibrils.45
The walls of microvessels are composed of an endothelial cell layer, basal lamina derived from extracellular matrix, and layers of SMCs and adventitia.13
The resistance provided by a small artery is a function of the diameter-pressure relation. This compliance depends on the amount, arrangement, and characteristics of the connective tissues and SMCs and on the activation level of SMCs.45
Coronary Arteries
A special mention should be made of the coronary artery wall, given the prevalence and importance of coronary artery disease with regard to diagnosis and treatment in patients undergoing vascular surgery. As is generally the case, coronary arteries have three concentric tunicae; however, several specialized features exist as well. The adventitia is thicker in proportion to other muscular arteries and includes a mix of collagen, elastic fibers, as well as adipose tissue. Collagen predominates in the adventitia compared with elastic fibers, in order to provide greater tensile strength and low stretch. Richly innervated smooth muscle cells in the media and adventitia are bundled longitudinally and circumferentially to respond to the repeated deforming forces caused by the contraction of the heart. Branching sites in the arteries also show normal, periodic thickenings of intima, termed musculoelastic cushions, that may contribute to atherosclerosis in these areas.48
Arterioles
Arterioles are the chief source of vascular resistance and control the distribution of flow. Abnormal restriction and dilatation cause systemic hypertension and hypotension, respectively. The smooth muscle of arterioles and, to some extent, that of small muscular arteries regulate blood flow to target tissues.
Small arteries and arterioles are controlled by both sympathetic and parasympathetic innervation. The response of small arteries and arterioles to neurohumoral activation under normal conditions largely depends on the type of stimulus and the arterial bed. Vasomotor responses depend on arteriolar territory, as in the case of emotional stress. Emotional stress dilates arterioles of skeletal muscles through activation of cholinergic vasodilator fibers and release of epinephrine while it constricts the splanchnic and renal arterioles.5 In larger arteries, emotional stress increases arterial pressure and blood flow velocity in large arteries but does not change arterial diameter.5,49
Capillaries
Capillaries are responsible for the transfer of nutrient materials and waste products between blood and tissues. The low rate of blood flow and large surface area facilitate the provision of nutrients and oxygen to surrounding tissue, the absorption of nutrients, waste products, and carbon dioxide, and the excretion of waste products from the body.
The surface area and blood volume of all capillaries are significantly larger than those of the aorta, causing a decrease in blood pressure and flow rate. The capillaries have a very large surface-area-to-volume ratio, allowing for nutrients to cross the capillary wall. Diameter ranges from 4 to 15 µm. Only the tunica intima is present in capillaries and typically only consists of endothelium, its basal lamina, and an incomplete layer of cells surrounding the capillary called pericytes. Pericytes have contractile properties and are able to regulate blood flow in the capillaries. During vascular remodeling and repair, pericytes are able to differentiate into ECs and SMCs.
There are three types of capillaries: continuous, fenestrated, and discontinuous. Each of these configurations has a different distribution within and between organs, allowing it to perform specific functions.
Continuous
A continuous EC and basal lamina function as selective filters, which allow substances to pass through the capillary wall without passing through both the EC and the basal lamina. For highly selective microcirculations such as the brain, with its tight blood-brain barrier, luminal and abluminal plasma membranes fused only at the tight junctions dictate the vascular permeability.50
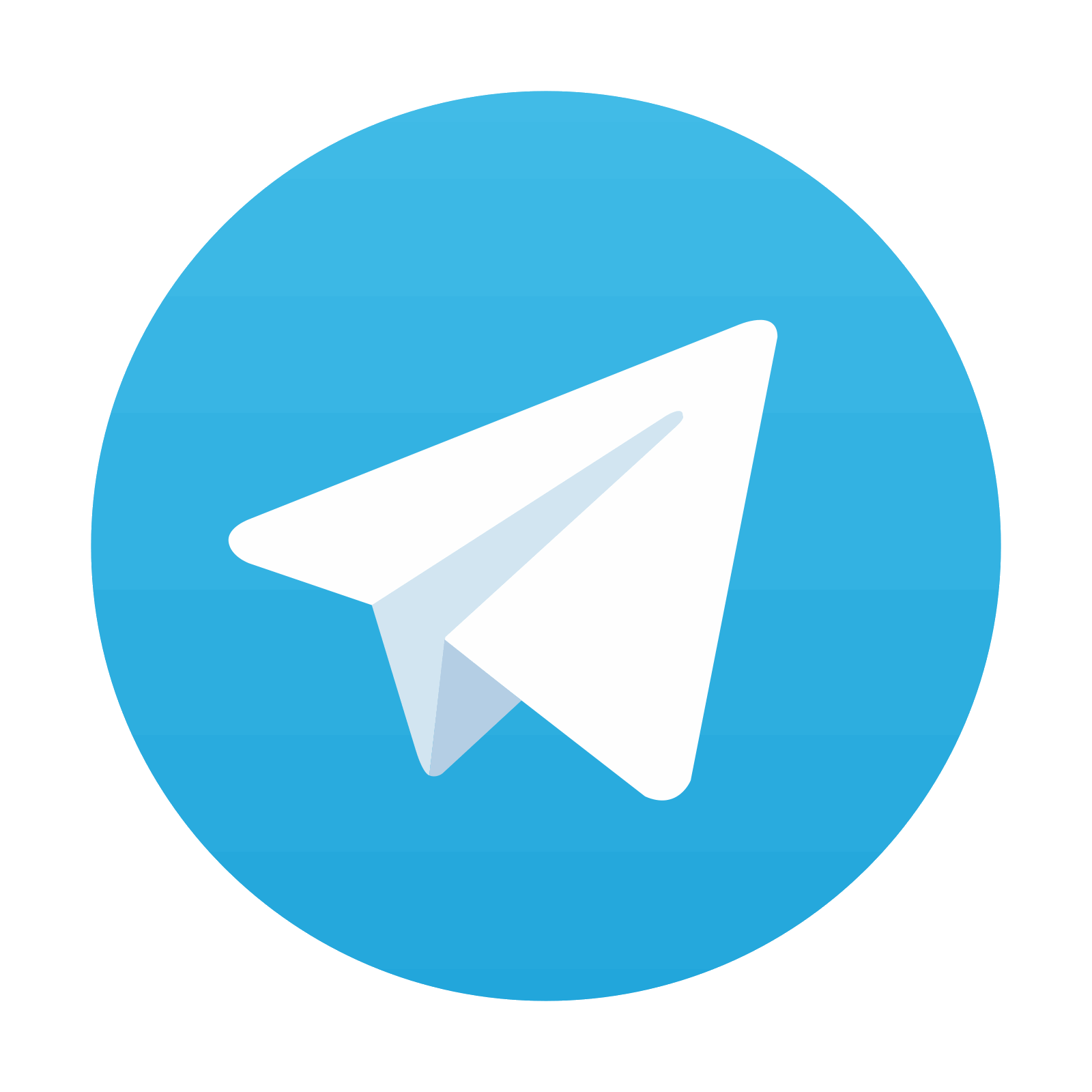
Stay updated, free articles. Join our Telegram channel
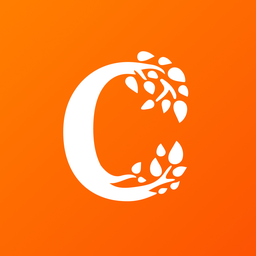
Full access? Get Clinical Tree
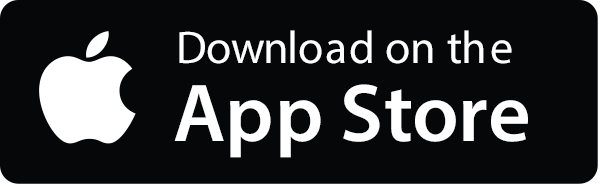
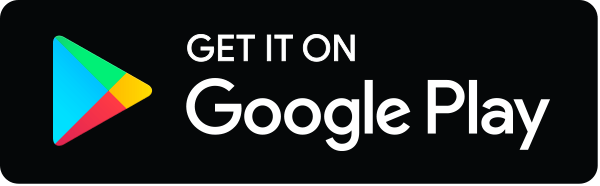