Constantine Mavroudis1, Carl L. Backer2, and Robert H. Anderson3 1Peyton Manning Children’s Hospital, Indianapolis, IN, USA 2UK Healthcare Kentucky Children’s Hospital, Lexington, KY, USA 3Newcastle University, Newcastle upon Tyne, UK A ventricular septal defect (VSD) is a hole between the ventricles. Such holes can be located anywhere in the normal ventricular septum, but can also exist at sites where, in the normal heart, there are no septal structures, such as directly beneath the leaflets of the pulmonary valve. They are characterized into anatomic types based on their location relative to the components of the right ventricle and their anatomic borders. The holes may vary in size, may be single or multiple, and may be associated with a number of other intracardiac and extracardiac anomalies. In this chapter, we discuss the management of isolated VSDs and those associated with persistent patency of the arterial duct, coarctation of the aorta, and acquired aortic insufficiency. Other associated major anomalies involving the ventricular outflow tracts, such as the defects seen in the setting of tetralogy of Fallot, double‐outlet right ventricle, common arterial trunk (truncus arteriosus), and transposition, are discussed elsewhere in the appropriate chapters. Also in other chapters are discussions of those defects associated with atrioventricular anomalies and those with discordant atrioventricular connections. The most common form of congenital heart disease in childhood is VSD, which may affect 0.5% of the population [1–6]. From January to December 2008, the Nationwide Inpatient Sample, the largest available inpatient care database in the United States containing 8 million hospital stays each year, registered 13,093 cases of congenital heart disease among 1,204,887 live births, with 31.4% of congenital heart disease cases being VSD [7]. In a population‐based cohort study in greater Paris, between May 2005 and April 2008, 52% of the 2867 neonates born with congenital heart disease were diagnosed with VSD [8]. In women with congenital heart disease, offspring complications are related to maternal cardiac function, especially those with maternal cyanosis or reduced cardiac output [9–11]. The overall recurrence rate of heart defects for offspring with maternal congenital heart disease is 6%; and the risk of VSD recurrence in offspring of a mother with congenital heart disease is also 6% [10]. Fesslova and associates studied the recurrence of congenital heart disease in pregnant women with familial risk [12]. Of the total 65 infants (21.5%) born with congenital heart disease, 14 were diagnosed with VSD, indicating a genetic component. Williams and colleagues found that maternal moderate to high alcohol consumption was associated with isolated VSDs [13]. Further, there was a twofold increase in the risk for isolated simple VSD for maternal marijuana use. Recent epidemiology perspectives have shown that maternal epilepsy (treated with carbamazepine), migraine, chronic hypertension, and paroxysmal supraventricular tachycardia may be associated with higher risk of VSDs, supported by a study of 3562 live‐born cases with congenital heart disease compared with neonates born without any birth defects or with other isolated congenital abnormalities [14]. The clinical manifestations attributed to VSD were first described by Roger in 1879 [15]. Eisenmenger [16], when studying the heart of a 32‐year‐old cyanotic patient, noted the presence of a large VSD with an overriding aorta, but in the absence of subpulmonary stenosis. It was much later that Abbott [17] and Selzer [18] formulated the pathophysiologic features of the VSD. The era of surgical intervention began in 1952, when Muller and Dammann placed a band on the pulmonary trunk to limit the flow of blood to the lungs in a patient with a VSD [19]. The first operative repair was performed by Lillehei and associates in 1954 [20]. They used controlled cross‐circulation with an adult acting as the pump oxygenator. The development of the heart–lung machine and cardiopulmonary bypass led rapidly to more widespread successful clinical results. DuShane and associates [21] had already shown by 1956 that transventricular repair could be performed with an acceptable mortality rate, while Stirling and coworkers [22] showed that closure could be accomplished transatrially, thereby avoiding a ventriculotomy. In 1961, Kirklin and associates [23], and later Sigmann and associates [24], reported on the successful repair in infants, an approach that in the majority of cases obviated the need for palliative banding. Later reports by Okamoto (1969) [25] and Barratt‐Boyes and colleagues (1976) [26] established the feasibility of repair in infants using deep hypothermia and circulatory arrest. Since that time, multiple refinements in methods of cardiopulmonary bypass and myocardial protection have allowed surgeons the time to perform accurate closure without incremental risk to the patient [27]. These advances have resulted in excellent short‐ and long‐term clinical results. An interventricular communication is an integral part of the pathway of circulation through the developing heart during the sixth to eighth weeks of fetal life. Subsequent to ventricular looping, the hole has the crest of the developing muscular ventricular septum as its floor, with the inner heart curvature forming its roof (Figure 16.1A). At this early stage, the developing heart exhibits a double inlet to the developing left ventricle, and a double outlet from the developing right ventricle. With expansion of the atrioventricular canal, the right ventricle acquires its inlet, but during this intermediate stage of development the separating arterial roots remain positioned above the right ventricular cavity (Figure 16.1B). At this intermediate stage of development, therefore, the interventricular communication serves as the outlet for the left ventricle. It is only after transfer of the subaortic outlet to the developing left ventricle that it becomes possible to close the interventricular communication. This is achieved by growth of tissue from the ventricular aspect of the atrioventricular cushions, with these components subsequently transformed to become the membranous part of the septum (Figure 16.1C). Knowledge of inappropriate completion of each of these developmental processes, namely transfer of the right ventricular inlet, separation of the arterial roots with transfer of the aortic component to the left ventricle, and eventual closure of the embryonic interventricular communication, combined with appreciation that the muscular septum itself is formed by compaction of the contained cardiomyocytes, now provides a unifying theme on which to base the categorization of the various types of septal defects that are to be encountered in postnatal life. The need for consensus when describing these lesions is well recognized, since the holes between the ventricles, even when seen in isolation, can show bewildering variation in their morphology. Over time, the defects have been defined and interpreted in different fashions by many authors [28–31]. One well‐respected surgical classification accounted for four types of defects in a numeric way (Figure 16.2) [32, 33]. We will endeavor to simplify this scheme by presenting an approach that highlights the phenotypic variability assessed on the basis of the anatomic borders of the defects. This permits us to condense the categories to three types. We do not propose to ignore the equally important feature of the location of the defect relative to the landmarks of the right ventricle, nor septal malalignment (Table 16.1). As will become evident, our suggested hierarchy is but a minimal departure from the initial numeric surgical classification. The numeric classification proposed four classes, but combined features of geography and borders when defining the subtypes. When concentrating on the phenotypic features as based on the anatomic borders, these can be reduced to three, namely the defects that are directly juxtapulmonary, those that are perimembranous, and those with exclusively muscular borders when viewed from the right ventricle. The specificity provided by concentration on these phenotypic features reinforces the simplicity of the initial approach. Our modifications are suggested to provide for the needs of modern surgery. This is because the provision of information relative to the borders of the defect permits inferences to be made, with considerable accuracy, regarding the likely location of the atrioventricular conduction axis. The “type I” defect as defined in the initial classification was a defect opening to the outlet of the right ventricle immediately beneath the pulmonary valve. Its phenotypic feature is fibrous continuity between the leaflets of the aortic and pulmonary valves in the roof of the defect due to failure of muscularization of the subpulmonary infundibulum. For this reason, the defect is considered by some as representing conal hypoplasia [31]. Indeed, it is frequently possible to recognize a fibrous raphe in the area of fibrous continuity between the arterial valves. It is the hypoplastic outlet septum and can be malaligned in either cranial or caudal direction, potentially obstructing the subpulmonary or subaortic outflow tracts, respectively. Because of failure of formation of the subpulmonary infundibular sleeve, the defect opens to the right ventricle between the limbs of the septal band (Figure 16.3). Figure 16.1 The images, prepared from episcopic datasets obtained from developing mouse embryos, show the changing morphology of the embryonic interventricular communication. (A) At embryonic day 10.5, the developing atrial chambers communicate only with the developing left ventricle, with the outflow tract supported exclusively above the developing right ventricle. The interventricular communication (double‐headed white arrow), therefore, is an integral part of the circulation. (B) It remains an integral part of the circulation at embryonic day 12.5, by which time the atrioventricular canal has expanded to produce the inlet of the right ventricle, containing the developing tricuspid valve, but with both outflow tracts remaining supported by the right ventricle, with the proximal parts of the outflow cushions still to fuse. (C) It is only subsequent to transfer of the aortic root, during embryonic day 13.5, that the embryonic interventricular communication is closed by growth of tissue from the ventricular aspect of the atrioventricular cushions. By this stage the proximal outflow cushions have fused with each other, with the septal cushion also fusing with the crest of the muscular ventricular septum, thus committing the aortic root to the left ventricle. Note that the trabeculations of the right ventricle are compacting to form the septal band. Figure 16.2 The four types of ventricular septal defect recognized in the scheme of classification favored for some time by the surgical community. (A) The “type I” defect, which is directly adjacent cranially to the leaflets of the tricuspid valve. (B) The “type II” defect opens centrally to the right ventricle at the base of the ventricular mass. (C) The “type III” defect is a large defect opening to the inlet of the right ventricle, and extending across the full width of the annulus of the tricuspid valve. (D) Several “type IV” defects, which open apically and are surrounded by the musculature of the ventricular septum. Source: Reproduced by permission from Mavroudis C et al., in Pediatric Cardiac Surgery, 3rd ed., Philadelphia, PA: Mosby; 2003, pp. 298–320. Table 16.1 Surgical approach to ventricular septal defects. Figure 16.3 The left‐hand panel shows the phenotypic feature of the “type 1” ventricular septal defect. It is directly juxtapulmonary, but also doubly committed because of its phenotypic feature, fibrous continuity between the leaflets of the aortic and pulmonary valves due to failure of formation of the free‐standing subpulmonary infundibular sleeve. In most instances, as shown, the defect has a muscular posteroinferior rim. The drawing in the right‐hand panel shows how this muscular rim protects the atrioventricular conduction axis. In some instances, however, the defect can extend so as to open centrally, when it is also perimembranous. Ao, aorta; AV, atrioventricular; IVC, inferior caval vein; PA, pulmonary artery; RA, right atrium; SVC, superior caval vein; TV, tricuspid valve; VSD, ventricular septal defect. Source: Reproduced by permission from Mavroudis C et al. (eds.), Atlas of Adult Congenital Heart Surgery, Cham: Springer Nature; 2020. In some of the classifications, the defect was considered to be “supracristal” [28]. As shown in Figure 16.3, the defect opens to the right ventricle between the limbs of the septomarginal trabeculation, or septal band. This feature, however, was taken to be the defining feature of the so‐called infracristal defect [28]. Thus, in this system of classification it was the structure taken to represent the “crista” that changed, rather than the location of the defect itself. The defect is better described as being doubly committed and juxtapulmonary. In the majority of cases, its posteroinferior rim is muscular, formed by fusion between the caudal limb of the septal band and the ventriculo‐infundibular fold. The muscle bar thus formed protects the atrioventricular conduction axis (Figure 16.3, right‐hand panel). In a minority of cases, the defect can extend to the central area of the ventricular base. It is then bordered posteroinferiorly by an area of fibrous continuity between the leaflets of the aortic and tricuspid valves. This is the phenotypic feature of the “type II” defect in the original surgical classification. It is of major surgical significance, since the conduction axis is at greater risk when the defect extends to reach the area of aortic‐to‐tricuspid fibrous continuity. It is the so‐called type II defect that is encountered most frequently during cardiac surgery. It exists because of failure to close the embryonic interventricular communication and opens to the right ventricle at the center of the cardiac base (Figure 16.4, left‐hand panel). The atrial component of the membranous septum is incorporated within this fibrous border (Figure 16.4, right‐hand panel). The defect was initially interpreted on the basis of failure of formation of the membranous septum. Hence, it was considered a “membranous” defect [28]. Nonetheless, a remnant of the interventricular part of the membranous septum, known as the membranous flap, is frequently found reinforcing the posteroinferior margin of the defect. The defect does not exist, therefore, because of absence of the membranous septum, but rather because of deficiency of its muscular margins. It was for this reason that it was described as being perimembranous [29]. Depending on the deficiency of its muscular borders, the central defect can also extend to open more to the inlet of the right ventricle than being beneath the septal leaflet of the tricuspid valve (Figure 16.5). Figure 16.4 The left‐hand image shows a defect opening centrally at the base of the right ventricle. As shown in the right‐hand diagram, its phenotypic feature is fibrous continuity at its posteroinferior margin between the leaflets of the aortic valve and the septal leaflet of the tricuspid valve. It opens inferior and posterior to the caudal limb of the septomarginal trabeculation, and behind the septal leaflet of the tricuspid valve, with the supraventricular crest inserted in normal fashion between the limbs of the trabeculation. As shown, the atrioventricular conduction axis runs posteroinferior to the defect but is at risk in this corner. Ao, aorta; AV, atrioventricular; IVC, inferior caval vein; PA, pulmonary artery; RA, right atrium; SVC, superior caval vein; TV, tricuspid valve; VSD, ventricular septal defect. Source: Reproduced by permission from Mavroudis C et al. (eds.), Atlas of Adult Congenital Heart Surgery, Cham: Springer Nature; 2020. Defects incorporating a deficiency at the central part of the ventricular base at the site of the embryonic interventricular communication can also be found extending to the outlet of the right ventricle. Such defects retain the phenotypic feature of fibrous continuity between the leaflets of the aortic and tricuspid valve, and hence are still perimembranous. They have the additional phenotypic feature of separation between the subpulmonary infundibulum and the crest of the muscular septum by virtue of malalignment of the muscular outlet septum. As with the fibrous outlet septum, there can be either cranial or caudal malalignment. It is cranial malalignment that is most frequent, in association with overriding of the aortic root, producing the lesion also known as the “Eisenmenger” defect (Figure 16.6). It is in this setting that the muscular outlet septum becomes recognizable as forming the cranial margin of the defect. This defect is described by some as being conoventricular [31]. Its right ventricular opening, like the juxtapulmonary or “type I” defect, is cradled between the limbs of the septal band. In this arrangement, the fibrous continuity forming the posteroinferior margin is between the leaflets of the aortic valve and the anterosuperior, rather than the septal, leaflet of the tricuspid valve [34]. In terms of its geography, it is an outlet defect, and hence could also be classified as representing a “type I” defect by those using the initial surgical classification. Like the central defect, nonetheless, and also the central defect extending to open to the inlet of the right ventricle, the defect extending to open to the right ventricular outlet shares the feature of fibrous continuity between the leaflets of the aortic and tricuspid valves, and hence is perimembranous. It is because of this feature that the atrioventricular conduction axis again penetrates directly through the posteroinferior border of the defect and is at risk of damage during surgical closure. Figure 16.5 The left‐hand panel shows a four‐chamber section through a heart with a defect located centrally within the ventricular base, but extending inferiorly so as to open to the inlet of the right ventricle, being shielded by the septal leaflet of the tricuspid valve. Since the defect retains the phenotypic feature of fibrous continuity between the leaflets of the aortic valve and the septal leaflet of the tricuspid valve, the conduction axis continues to run posteroinferiorly relative to the defect when viewed from the right ventricle (right‐hand panel). Ao, aorta; AV, atrioventricular; IVC, inferior caval vein; PA, pulmonary artery; RA, right atrium; SVC, superior caval vein; TV, tricuspid valve; VSD, ventricular septal defect. Source: Reproduced by permission from Mavroudis C et al. (eds.), Atlas of Adult Congenital Heart Surgery, Cham: Springer Nature; 2020. Figure 16.6 The left‐hand panel shows a defect with malalignment of the muscular outlet septum and associated overriding of the aortic root. It extends centrally to become perimembranous and is the so‐called Eisenmenger defect. As shown in the right‐hand panel, because the defect extends to become perimembranous, the conduction axis continues to extend posteroinferiorly relative to the margins of the defect. Ao, aorta; AV, atrioventricular; IVC, inferior caval vein; PA, pulmonary artery; RA, right atrium; SVC, superior caval vein; TV, tricuspid valve; VSD, ventricular septal defect. Source: Reproduced by permission from Mavroudis C et al. (eds.), Atlas of Adult Congenital Heart Surgery, Cham: Springer Nature; 2020. The lesion in the initial surgical classification described as the “type III” defect was considered to represent an inlet defect. It was described in this fashion because of its geographic location relative to the landmarks of the right ventricle. As we have already established, however, the perimembranous defect can open to the inlet of the right ventricle when associated with deficiency of the inferior and basal part of the muscular ventricular septum (Figure 16.5). In fact, the phenotypic feature of the lesion accorded separate status as the “type III” defect is malalignment between the apical muscular septum and the atrial septum (Figure 16.7, left‐hand panel). It exists because of incomplete expansion of the atrioventricular canal during embryonic development. Because of the abnormal development of the inlet component, there is overriding and straddling of the tricuspid valve [35]. By virtue of this feature, the malaligned ventricular septum extends across the full circumference of the tricuspid valvar orifice (Figure 16.7, right‐hand panel). This appearance is similar to that seen in atrioventricular septal defect with common valvar orifice. It was this finding that presumably prompted some investigators to categorize the lesion as an “atrioventricular canal defect” [31]. Patients with straddling and overriding tricuspid valve, however, do not exhibit a common atrioventricular junction, which is the essence of an “atrioventricular canal.” In a small minority of patients having an atrioventricular septal defect in the setting of a common atrioventricular junction, in other words an unequivocal atrioventricular canal, it is possible for shunting across the defect to be confined at the ventricular level, because the bridging leaflets of the common valve are attached to the leading edge of the atrial septum (Figure 16.8). Figure 16.7 The image in the left‐hand panel shows a defect unequivocally opening to the inlet of the right ventricle. Its phenotypic feature, however, is malalignment of the muscular ventricular septum relative to the atrial septum, with straddling and overriding of the tricuspid valve. As is shown in the drawing in the right‐hand panel, because of the malalignment of the muscular septum, which carries the atrioventricular conduction axis, the axis arises from an anomalous atrioventricular node formed at the point where the malaligned ventricular septum meets the inferior component of the atrioventricular junction. The regular atrioventricular node is hypoplastic but remains in its expected position within the triangle of Koch. Ao, aorta; AV, atrioventricular; IVC, inferior caval vein; PA, pulmonary artery; RA, right atrium; SVC, superior caval vein; TV, tricuspid valve; VSD, ventricular septal defect. Source: Reproduced by permission from Mavroudis C et al. (eds.), Atlas of Adult Congenital Heart Surgery, Cham: Springer Nature; 2020. These lesions found with common atrioventricular junctions are the true defects of “atrioventricular canal type” that produce ventricular shunting. We have considered the details of these malformations in greater detail in Chapter 15. This is because the hole is the ventricular component of an atrioventricular septal defect, rather than representing a VSD, as is the case for the lesions to be discussed in this chapter. In the lesion with overriding of the tricuspid valvar orifice, or the initial “type III” defect, it is the malalignment between the atrial septum and the muscular ventricular septum that is the major phenotypic finding (Figure 16.7, left‐hand panel). Because of septal malalignment, the atrioventricular conduction axis no longer takes its origin from the regular node located at the apex of the triangle of Koch (Figure 16.7, right‐hand panel). Instead, it arises from an anomalously located atrioventricular node. This important surgical feature reflects the phenotype rather than the geography of the defect. And, as we have already shown, other defects with different phenotypic features can open to the inlet of the right ventricle, such as the central perimembranous defect with inlet extension (Figure 16.5), or the atrioventricular septal defect with exclusively ventricular shunting (Figure 16.8). It follows, therefore, that to provide the specificity required for surgical description, it is necessary to account not only for the geography but also the phenotypic features of the various types of VSD. This is the more important issue when considering the defects that open to the inlet of the right ventricle, since defects with exclusively muscular borders can share this feature (Figure 16.9, left‐hand panel). And, when the defect opening to the right ventricular inlet has exclusively muscular borders, the conduction axis is at risk anterosuperiorly, or to the left hand of the surgeon operating through the tricuspid valve (Figure 16.9, right‐hand panel) Figure 16.8 The features of an atrioventricular septal defect with exclusively ventricular shunting, by virtue of the bridging leaflets of the common valve being attached to the leading edge of the atrial septum. In this setting, the conduction axis arises from an anomalous node located at the cardiac crux, rather than at the apex of the triangle of Koch. AV, atrioventricular; VSD, ventricular septal defect. Source: Reproduced by permission from Mavroudis C et al., in Pediatric Cardiac Surgery, 4th ed., Chichester: Wiley‐Blackwell; 2013, pp. 311–341. Figure 16.9 The heart shown in the left‐hand panel has a defect opening to the inlet of the right ventricle, being seen subsequent to retraction of the septal leaflet of the tricuspid valve. In this heart, however, the defect has exclusively muscular borders. This means that the conduction axis will be located anterosuperiorly relative to the defect. As shown in the right‐hand panel, the axis will be to the left hand of the surgeon working in the operating room. Source: Reproduced by permission from Mavroudis C et al. (eds.), Atlas of Adult Congenital Heart Surgery, Cham: Springer Nature; 2020. In terms of geography, therefore, the central perimembranous defect extending inferiorly is also an inlet defect (Figure 16.10, left‐hand panel). With this variant, however, unlike the so‐called type III defect (Figure 16.10, right‐hand panel), the atrioventricular conduction axis retains its anticipated origin from the atrioventricular node located at the apex of the triangle of Koch, although both triangle and node are deviated inferiorly within the right atrium. Figure 16.10 The drawings show the location of the conduction axis in the setting of defects extending inferiorly from the central perimembranous area so as to open primarily to the inlet of the right ventricle, but in the settings of atrioventricular septal alignment (left‐hand panel) and atrioventricular septal malalignment (right‐hand panel). When the septal components are aligned, as shown to the left, the atrioventricular node remains located at the apex of the triangle of Koch, but the triangle, and the node, are displaced inferiorly, although not located at the cardiac crux, as is the case for the atrioventricular septal defect with exclusive ventricular shunting (compare with Figure 16.8). When there is septal malalignment, in contrast, the conduction axis arises from an anomalous atrioventricular node (right‐hand panel). Source: Reproduced by permission from Mavroudis C et al., in Pediatric Cardiac Surgery, 4th ed., Chichester: Wiley‐Blackwell; 2013, pp. 311–341. Since the defect found with straddling and overriding of the tricuspid valve is also associated with fibrous continuity between the leaflets of the aortic and tricuspid valves, it remains a perimembranous defect, but with the additional essential feature of malalignment between the atrial septum and the muscular ventricular septum. The unifying feature of the final types of defect, which were classified as being “type IV” in the initial system, is that they all have exclusively muscular borders (Figure 16.11). They can show marked variability, however, in terms of their geography. As we have already described, some open to the inlet of the right ventricle (Figure 16.9), which might justify their classification as “type III” defects. Others are found opening to the outlet of the right ventricle, making them describable as “type I” defects, the more so since they open between the limbs of the septal band (Figure 16.12). In terms of classification, they are well described as muscular outlet defects. The muscular outlet defects exist because of separation between the muscular septum and the subpulmonary infundibulum, and hence can also be considered as representing conal hypoplasia. Muscular defects, moreover, can also be found at various locations within the basal, middle, or apical part of the muscular ventricular septum, and can be located anteriorly or posteriorly relative to the septal band. The latter lesions are produced because of incomplete compaction of the developing apical part of the septum [36, 37]. Larger muscular defects can be crossed by right ventricular trabeculations, such that a solitary defect as viewed from the left ventricular aspect seemingly has multiple orifices when viewed from the right ventricle. There can also be multiple small defects percolating through the apical septum, producing the arrangement known as the Swiss‐cheese septum. Any of the muscular defects can also coexist with perimembranous defects. If the muscular defect in such a combination opens to the inlet of the right ventricle, the conduction axis will run within the muscular strand interposing between the defects. This can be very thin, making it difficult to close the defects independently without producing atrioventricular block. A defect that was not included in the initial numeric classification is the Gerbode defect. This is a direct communication between the left ventricle and the right atrium. It can take two forms. In the majority of cases, the ventriculo‐atrial shunting is through a central VSD associated with deficiency of the septal leaflet of the tricuspid valve. Much more rarely, the lesion represents a congenital deficiency of the atrioventricular component of the membranous septum (Figure 16.13). Despite the rarity of the latter form, often considered to be the “true” Gerbode defect, six examples have been repaired over a period of 18 years at Ann & Robert H. Lurie Children’s Hospital of Chicago [38]. They are atrioventricular septal defects, but in the setting of separate right and left atrioventricular junctions. We consider them here for the sake of completeness, but they could also have been placed in Chapter 15. It is the absence of tricuspid regurgitation that distinguishes the true Gerbode defect from the indirect forms. The latter variants, as indicated, are central VSDs associated with deficiencies in the leaflets of the tricuspid valve. Spontaneous closure is very rare in either instance, so surgical closure is recommended, and is associated with excellent results. Figure 16.11 The drawing shows how defects with exclusively muscular borders, defined as “type IV” in the initial surgical classification, can open to any part of the morphologic right ventricle. This means that, as shown, they vary with regard to their relationship to the atrioventricular conduction axis. Ao, aorta; AV, atrioventricular; IVC, inferior caval vein; PA, pulmonary artery; RA, right atrium; SVC, superior caval vein; TV, tricuspid valve. Source: Reproduced by permission from Mavroudis C et al. (eds.), Atlas of Adult Congenital Heart Surgery, Cham: Springer Nature; 2020. Any heart having deficient ventricular septation can be associated with further additional lesions, of which the most important are obstruction of the ventricular outflow tracts and prolapse of the leaflets of the aortic valve. Aortic valvar prolapse, leading to valvar regurgitation, is found most usually with either the doubly committed and juxtaarterial defects, or with malalignment outlet defects, with or without perimembranous extension. Outflow tract obstruction most usually reflects malalignment of the outlet septum, be it muscular or fibrous. Anterocephalad malalignment is the essence of tetralogy of Fallot, which has its own chapter in this book (Chapter 21). Posterocaudal malalignment, in contrast, produces subaortic obstruction. This is typically associated with either severe aortic coarctation or interruption of the aortic arch. Our suggested unifying approach for categorization and description of VSDs, therefore, combines the features of location of the defect relative to the landmarks of the right ventricle, its phenotype defined on the basis of the anatomic nature of its borders, and the presence of septal malalignment. As we have shown, it is but a minimal departure from the initial numeric surgical classification. Its major feature is to use the borders of the defect as seen during surgical closure as the first step in the hierarchical classification. A hierarchy is required in any categorization, the more so if potential risk factors are to be identified by those who report their results to databases such as that maintained by the Society of Thoracic Surgeons and the Congenital Heart Surgeons’ Society. For the purposes of comparison of surgical results, we suggest that the hierarchy based on borders will have most utility, the more so since our modification includes all the information regarding geography and septal malalignment. Figure 16.12 The location of a muscular defect opening to the outlet of the right ventricle. It exists because of separation between the subpulmonary infundibular sleeve and the crest of the muscular ventricular septum. The posteroinferior muscular rim of the defect protects the atrioventricular conduction axis (yellow dotted line). Source: Reproduced by permission from Mavroudis C et al. (eds.), Atlas of Adult Congenital Heart Surgery, Cham: Springer Nature; 2020. It is the size of the VSD, along with the pulmonary vascular resistance, that determines the magnitude of the left‐to‐right shunt. Other factors include ventricular compliance and obstructions to pulmonary or aortic outflows. The physiologic effects are age dependent, being related early in life to pulmonary vascular resistance and later in life to the degree of narrowing and eventual closure of the VSD. For example, at birth pulmonary vascular resistance is predictably high and usually blunts the potential left‐to‐right shunt, to the extent that the diagnosis often may go unrecognized. Within weeks to months, the pulmonary vascular resistance falls, resulting in an increasing left‐to‐right shunt and audible murmur. When shunting is excessive, congestive heart failure develops, manifested by dyspnea, poor feeding, frequent upper respiratory infections, and failure to thrive. In these patients, pulmonary vascular changes may begin to develop as early as the first months of life with pulmonary arteriolar thickening [39]. Typically, after 2–3 years, these changes may progress, resulting in irreversible pulmonary vascular obstructive disease. In later stages, pulmonary vascular resistance may exceed systemic vascular resistance, resulting in reversal of the shunt, cyanosis, and eventual death. Figure 16.13 The morphology of the so‐called direct Gerbode defect, which exists because of a deficiency of the atrioventricular component of the membranous septum. Children with restrictive defects and decreased left‐to‐right shunting usually remain asymptomatic with little or no medical therapy. They may improve symptomatically as the relative size of the VSD decreases. This leads to a clinical dilemma concerning the most prudent time for referral for surgical closure. Although the progression of pulmonary vascular disease is variable, it is generally agreed that irreversible pulmonary vascular disease will not occur before 1–2 years of age. The clinical course of patients born with a VSD is quite variable, depending to a large degree on the size of the defect, the magnitude of the left‐to‐right shunt, and the pulmonary vascular resistance. Most VSDs are restrictive, being less than 0.5 cm diameter. Of those with an isolated VSD, 80% will undergo spontaneous closure by the age of 1 month, obviating the need for surgical closure in the majority of cases [40]. The mechanisms of spontaneous closure include fibrosis of the margins induced by hemodynamic changes; adherence of the septal leaflet of the tricuspid valve to the defect, often forming a tricuspid tissue pouch; and muscular hypertrophy, which occurs most commonly with muscular defects [41]. The incidence of spontaneous closure is highest during the first year of life, but continues to a lesser degree up to approximately 5 years, after which spontaneous closure is rare [42]. An undesirable mechanism of spontaneous occlusion is prolapse of an aortic valvar leaflet into the defect, oftentimes causing aortic insufficiency. This is an indication for prompt surgical repair, albeit that it is preferable to identify the defects associated with this potential complication and close them before its development. If spontaneous closure does not occur in childhood, the chance that it will close in adulthood is small, reported to occur in only 10% of patients [40, 43]. With advancing age, symptoms resulting from living with a left‐to‐right shunt may lead to endocarditis, aortic regurgitation, arrhythmias, pulmonary hypertension, and heart failure [40]. In patients with larger VSDs, symptoms develop soon after birth, concomitant with the fall in the elevated neonatal pulmonary vascular resistance. Congestive heart failure, manifested by dyspnea, repeated pulmonary infections, hepatomegaly, sweating, and failure to thrive, usually responds to medical therapy, but may persist. Increased pulmonary vascular resistance secondary to hypertensive pulmonary vascular disease worsens with increasing age [44–46]. Patients are at risk of developing irreversible pulmonary vascular disease after 1–2 years of age. This process may lead to Eisenmenger syndrome, which is characterized by greater pulmonary than systemic vascular resistance, reversal of the ventricular shunt with resultant cyanosis, and eventual right ventricular failure. Although some patients show increased pulmonary vascular tone in the first year of life, Eisenmenger syndrome typically takes much longer to develop, appearing most frequently in the second and third decades of life and typically leading to death by the age of 40 [47]. Some children with isolated defects develop subpulmonic stenosis due to right ventricular infundibular hypertrophy, producing physiology similar to tetralogy of Fallot. Because the pulmonary arteriolar beds have been protected from the high ventricular pressures, these patients are not at risk for pulmonary vascular destructive disease, in much the same way as if the patient had undergone palliative banding of the pulmonary trunk. Bacterial endocarditis is a rare complication and occurs at a rate of approximately 0.3% per year for each patient with a VSD [48–53]. Recent evidence, however, shows that infective endocarditis in adults followed by unoperated VSDs is higher than previously thought [53]. Its development is indicated by fever, bacteremia, and recurrent pulmonary infections. The locus of infection is usually the septal leaflet of the tricuspid valve, which may result in an altered systolic murmur and localized vegetations identifiable by echocardiography. Antibiotic treatment is usually effective in controlling the active infection. After such an episode, closure of the VSD, regardless of its size, and tricuspid valve repair are indicated (see Chapter 45). Prophylactic closure of restrictive VSDs in the absence of traditional indications for closure remains controversial. This is because of the small incidence of endocarditis compared with the small, but nevertheless present, risk of morbidity and mortality from surgical closure, as well as emotional and cosmetic considerations. VSDs are often associated with other lesions that develop because of their anatomic and hemodynamic characteristics. Prolapse of the leaflets of the aortic valve occurs most frequently with the defects opening directly into the outlet of the right ventricle, be these defects doubly committed and juxtaarterial, muscular outlet, or perimembranous defects opening to the right ventricular outlet with malalignment of the muscular outlet septum. Either the right or noncoronary leaflets of the aortic valve can be involved. With advancing age, untreated prolapse of the aortic valvar leaflets results, first, in a decreased left‐to‐right shunt because the involved leaflet often prolapses into the defect and reduces the shunt and, second, worsening aortic insufficiency [27, 54–56]. Early surgical closure may prevent the development of aortic insufficiency in those patients who initially had prolapse without insufficiency. If aortic insufficiency is present, repair can be performed at the time of surgical closure. Chronic turbulence through the VSD can also lead to bacterial endocarditis, which most often affects the septal leaflet of the tricuspid valve [49]. Patients with restrictive VSDs are generally diagnosed by the discovery of a loud holosystolic murmur heard best at the left sternal border, rarely accompanied by a hyperactive precordium and a ventricular bulge. The intensity of the murmur may vary significantly and can be inversely related to the size of the defect. The smaller its size, the louder the murmur. As the pulmonary vascular resistance rises, the murmur becomes shorter, softer, and limited to the early part of systole. It may disappear entirely, leaving a loud second heart sound due to closure of the pulmonary valve and indicative of severe pulmonary hypertension. The liver may be enlarged, and the neck veins can be distended. Changes in chest x‐ray are relative to the size of the left‐to‐right shunt. They include varying degrees of increased pulmonary vascularity and cardiomegaly with biventricular enlargement (Figure 16.14) [56]. The electrocardiogram may be normal or may show right, left, or combined ventricular hypertrophy. Cross‐sectional echocardiography, coupled with Doppler interrogation, now permits cardiologists not only to diagnose the presence of VSDs, but also to establish their phenotype. Multiple views help determine the location and size of the VSD, its borders, the morphology of the ventricular outflow tracts, the involvement of the aortic valve, and the proximity to the tension apparatus of the atrioventricular valves (Figure 16.15) [56]. This technique, however, does not provide other important information that is determined by cardiac catheterization, such as quantification of ratio of systemic and pulmonary flows, the pulmonary arterial pressure, and accurate identification of multiple defects (Figure 16.16) (see Chapter 4) [56]. The current diagnostic trend, nonetheless, is away from cardiac catheterization, with greater reliance on echocardiographic and Doppler interrogation. The reason for this change is based on surgical trends toward earlier closure. For instance, a 3–6‐month‐old infant with a demonstrated large VSD and related symptoms almost assuredly has elevated pulmonary arterial pressures and a large left‐to‐right shunt. Moreover, the chance of irreversible pulmonary vascular disease approaches zero. Consequently, cardiac catheterization, while technically informative, does not impact on the decision to advocate surgical closure in light of the current advances in echocardiographic techniques. On the other hand, a child or young adult with a large VSD may have increased pulmonary vascular resistance, which cannot accurately be determined by echocardiography. In these circumstances cardiac catheterization is required to measure pulmonary arterial pressure, to calculate pulmonary vascular resistance, and to determine the response of the pulmonary arteries to vasodilators, all of which will influence the decision regarding surgical closure. Figure 16.14 Chest roentgenograph of a patient with a large ventricular septal defect, showing cardiomegaly and increased pulmonary vascularity due to the large left‐to‐right shunt. Source: Reproduced by permission from Mavroudis C et al., in Pediatric Cardiac Surgery, 3rd ed., Philadelphia, PA: Mosby; 2003, pp. 298–320. Figure 16.15 Echocardiographic views showing examples of the various types of ventricular septal defects (VSDs). (A) Parasternal long‐axis view showing a doubly committed and juxtaarterial VSD. Arrow indicates the VSD, with the right coronary leaflet of the aortic valve prolapsing into the defect. (B) Parasternal long‐axis view showing a perimembranous VSD. Arrow indicates tricuspid pouch (accessory tricuspid tissue). (C) Apical four‐chamber view showing a so‐called atrioventricular canal‐type VSD (inlet VSD). Arrow indicates the position of the VSD in relation to the mitral and tricuspid valves at the inlet portion of the right ventricle. Note that the patient does not have a common atrioventricular canal. (D) Apical four‐chamber view showing a mid‐muscular VSD. Arrow indicates the position of the VSD. AO, aorta; LA, left atrium; LV, left ventricle; RA, right atrium; RV, right ventricle. Source: Reproduced by permission from Mavroudis C et al., in Pediatric Cardiac Surgery, 3rd ed., Philadelphia, PA: Mosby; 2003, pp. 298–320. Medical management is directed at the pathophysiologic consequences of the left‐to‐right shunt, the management of increased pulmonary vascular resistance, and prophylactic antibiotic administration for endocarditis. Infants with congestive heart failure often respond to digitalis, diuretic therapy, and afterload reduction. Supplemental nutritional support may be necessary because of poor feeding and failure to thrive. Optimal antibiotic therapy will be required for recurrent pulmonary infections. These supportive measures often allow delay of surgical treatment and may promote spontaneous restriction or closure of the VSD. When these measures are not effective, surgical closure should be undertaken. Occasionally, a patient may require assisted ventilation and inotropic support to treat decompensated congestive heart failure as a bridge to surgical therapy. Under these conditions, a thorough evaluation should be undertaken to determine causes for the decompensation, such as subaortic stenosis, coarctation, persistent patency of the arterial duct, or infection. Figure 16.16 Left ventriculograms demonstrating various types of ventricular septal defect (VSD). (A) Doubly committed and juxtaarterial VSD (conal, supracristal, subarterial). (B) Perimembranous (arrow indicates prolapse of the aortic valve through the VSD). (C) Inlet type. (D) Midmuscular VSD. (E) Multiple muscular VSD, “Swiss‐cheese” type. Source: Reproduced by permission from Mavroudis C et al., in Pediatric Cardiac Surgery, 3rd ed., Philadelphia, PA: Mosby; 2003, pp. 298–320. Older patients with pulmonary hypertension and elevated pulmonary vascular resistance require cardiac catheterization to determine the pulmonary arterial pressure, along with the response to inspired oxygen and other vasodilators such as amrinone, isoproterenol, nitroglycerin, nitroprusside, inhaled nitric oxide, and prostaglandin E1. An increase in left‐to‐right shunting and/or a decrease in the mean pulmonary arterial pressure are considered responses favoring VSD closure. These same agents can be administered postoperatively to promote resolution of reversible pulmonary hypertension. The selection of patients for VSD closure centers around the size and type of the defect as determined by echocardiography and/or cardiac catheterization, the natural history, the intervening symptoms caused by the VSD, and complicating factors. Infants with large VSDs and severe, intractable congestive heart failure should undergo surgical closure at any time during the first 3 months of life. If, however, these patients respond to medical therapy, then conservative follow‐up is warranted up to about 6 months of age. Spontaneous closure of large VSDs becomes less probable after this time (Figure 16.17) [27, 56–60], while the incidence of progressive pulmonary vascular obstructive disease increases. Closure should be undertaken at this time, especially if the pulmonary vascular resistance is high, greater than 4 units/m2 [57, 58], or if the ratio of pulmonary to systemic flows exceeds 2 : 1. Older patients with large VSDs occasionally present with varying degrees of established pulmonary vascular obstructive disease. Predictably, these patients have a history of some antecedent clinical improvement due to the decreased left‐to‐right shunt in response to the increased pulmonary vascular resistance. Patients with a dominant right‐to‐left shunt and calculated pulmonary vascular resistance higher than 8 units/m2 who show no response to pulmonary arterial vasodilatation have not been considered candidates for surgical closure in the past. Some centers have depended on lung biopsy to evaluate the degree of pulmonary vascular changes, but this has now fallen from favor [61–63]. Other centers have embarked on a staged medical‐surgical therapy to include preoperative sildenafil or bosentan followed by VSD closure [64–75]. The symptoms of severe pulmonary arterial hypertension (PAH) resulting from VSD not corrected at an early stage include dyspnea, cyanosis, fatigue, dizziness, and syncope, and eventually reduced life expectancy (greater than a twofold risk of mortality and threefold risk of heart failure and arrhythmias) [64, 70, 71]. Figure 16.17 Probability of eventual spontaneous closure of a large ventricular septal defect (VSD) according to the age at which the patient is observed. The broken lines enclose the 70% confidence limits (CLs) around the solid probability line. The specific ratios, with the 70% CLs reported by Hoffman and Rudolph [59] (1966) and Keith and associates [58] (1971), are shown centered on the mean or assumed ages of patients in their reports (p<.001). Source: Reproduced by permission from Blackstone EH et al. J Thorac Cardiovasc Surg. 1976;72:661–679. Some therapies for advanced PAH have been proven to reduce pulmonary vascular resistance effectively and include PAH‐targeted drugs such as prostanoids (e.g., epoprostenol), endothelin receptor antagonists (e.g., bosentan, ambrisentan), and phosphodiesterase‐5 inhibitors (e.g., sildenafil, tadalafil) for patients who were previously thought to have irreversible pulmonary vascular disease [64, 65, 65–69]. These therapies are also being used preoperatively and postoperatively to reduce postoperative PAH [64, 73]. In one study, 56 patients with nonrestrictive VSD were treated with an advanced preoperative PAH therapy of oral sildenafil or bosentan for 1–2 months and then surgically repaired [64]. Patient records were reviewed retrospectively and there were no operative deaths, a significant increased baseline six‐minute walk test distance of all patients, and significant mean pulmonary artery pressure regression (p<.01). Another study by Hu and associates [71] used targeted sildenafil or bosentan (3–15 months preoperatively, average 7.61 ± 3.00 months) to decrease pulmonary circulation resistance before and after surgery (even early postoperatively) in 41 adult patients with VSD (average age 28.15 ± 8.14 years) [71, 72]. Pulmonary vascular resistance was significantly decreased after medical treatment (17.08 ± 3.97 vs. 12.76 ± 2.73) and surgical repair was completed. Two patients died during hospitalization (mortality 4.9%). A total of 39 patients were followed once every 6 months after discharge for an average of 37 months. Among them, 36 cases continued targeted drug therapy and their mean pulmonary artery pressure was significantly reduced. No patient died during follow‐up. Pulmonary hypertension due to delay in presentation, diagnosis, referral, and surgery for septal defects is not uncommon in the developing world and translates into high morbidity and mortality [76–81]. Children with large VSDs and pulmonary hypertensive events are rarely seen in surgically advanced countries, but do present a significant problem in the remainder of the world, where sophisticated pharmacologic agents such as sildenafil or nitric oxide or circulatory assist devices such as extracorporeal membrane oxygenation are not readily available. Zhou and colleagues first described unidirectional valved patch closure for VSD in patients with severe pulmonary artery hypertension in 1995, constructed from a Dacron patch of the approximate size of the defect and a 0.5–1.0 cm diameter fenestration somewhat off the center of the patch, in addition to the attachment of a piece of quadrangular pericardium with one unattached edge tailored to provide a small opening, not exceeding 0.5 cm, and to maintain tension on the pericardial edge to prevent a loose or incompetent valve [79, 81]. Novick and colleagues modified the fenestrated flap valve double VSD patch [77] constructed from Sauvage Dacron (C.R. Bard, Murray Hill, NJ, USA) and Gore‐Tex (W.L. Gore, Newark, DE, USA). The patch was tailored according to each individual defect and fenestration was made in the center of the patch. The fenestration size was determined by the size of each child, the diameter being one‐half of the expected aortic annulus. A separate flap patch at least 4 mm larger than the fenestration was then sewn onto the superior margin of the fenestration along one‐third of the circumference, and a tethering stitch was placed along the diameter of the fenestration. The patch was then sewn, leaving the flap valve on the left ventricular side directing the flap to open toward the left ventricular apex. Of the 91 children operated on using the Novick flap [77], 56 patients had primary VSD and 54 survived (96.4%), compared with 30 of the 35 complex VSD group (85.7%), confirming that the double‐patch technique provides a simple physiologic mechanism for unloading the right ventricle during periods of severe pulmonary hypertension whether acute, transient, or sustained. Rao and associates also found flap valved closure of VSD to decrease morbidity and mortality in 18 consecutive patients with a large VSD and severe pulmonary hypertension [76]. In‐hospital 30‐day mortality was 5.6% (1 of 18). Postoperative pulmonary artery pressures were significantly reduced. Four patients had pulmonary hypertensive crisis postoperatively. There were no late cardiac deaths. Talwar and colleagues also worked with a unidirectional valved patch [78]. After inspecting the VSD, a patch of knitted polyester fabric (Impra, Tempe, AZ, USA) was constructed in the same width as the defect and approximately 1.5 times longer than the desired length along with a 4 mm fenestration. The patch was then folded to achieve adequate dimensions and cover the fenestration to provide a valved mechanism. The patch was sutured to the edges of the septal defect to allow the flap to lie toward the left ventricular side and orient the flap to open downward toward the left ventricular apex, so as not to produce left ventricular outflow tract obstruction during systole. Talwar and colleagues have used the patch for VSD closure in 17 patients [78]. The shunt was bidirectional in 15 patients and predominantly right‐to‐left in 2 patients. Postoperative right‐to‐left shunt across the patch was found in 3 patients (2, 7, and 9 years old with preoperative indexed pulmonary vascular resistance of 9.5, 9.8, and 11.1 Woods units, respectively). There were no in‐hospital deaths. At a mean follow‐up of 30 ± 14.7 months, all patients were well without cyanosis. Echocardiography showed no shunt across the patch and all patients had systemic saturation >95%. There has been limited experience with VSD closure and single‐ or double‐lung transplantation for those patients with progressive pulmonary vascular disease. The results of this therapy are discussed in Chapter 44. Infants shown by echocardiography and physical examination to have small VSDs require little or no medical therapy. They are followed with the expectation that the VSDs may get smaller and/or undergo spontaneous closure. After the first year of life, asymptomatic patients with small perimembranous or muscular defects can be reevaluated on a timely basis for physiologic and anatomic signs that would suggest indications for surgical closure. Recent data have led to questions regarding the validity of historical indications for surgical closure [82, 83]. The proposed, more liberal indications are any evidence of aortic valvar prolapse (even without regurgitation), a history of prior endocarditis, and any evidence of ventricular dilation, even if the left‐to‐right shunt is less than 2 : 1. A previous report by Backer and colleagues from 1993 revealed 141 patients with a Qp : Qs ratio of less than 2 : 1 who had undergone surgical closure from 1980 to 1991 for one or more of the above indications [82]. There were no operative or late deaths, no instances of heart block, and no reoperations for residual shunting. Transatrial or transpulmonary repair was feasible in all. These data, although presently mildly controversial, suggest that the surgical risk of closing a restrictive VSD permitting a high‐velocity turbulent jet is less than the combined lifetime risk of bacterial endocarditis, aortic valvar prolapse that may lead to aortic insufficiency, and tricuspid regurgitation [83, 84]. These patients have a high probability of developing aortic valvar prolapse and aortic insufficiency, especially after 5 years of age (Figure 16.18) [56, 85]. Early surgical intervention may control or prevent the progression of aortic insufficiency [85–92]. Because of the known natural history and the impact of surgery, all doubly committed and juxtaarterial defects (conal or supracristal VSDs) should be closed regardless of the magnitude of the left‐to‐right shunt. Preoperative demonstration of the location of the VSD determines the options available with regard to the cardiac incisions needed for exposure, avoidance of injury to the conduction pathways, and the operative technique needed to secure closure when part of the perimeter of the defect is formed by the leaflets of the atrioventricular, pulmonary, or aortic valves. In general, there are five operative approaches for VSD closure: right atrial, transpulmonary, transaortic, right ventricular, and left ventricular. Complex and multiple VSDs, or those that have extensive involvement of more than one region of the septum, may require a combined approach. Figure 16.18 Onset curve of aortic insufficiency. Comparison of age versus probability of being free of aortic insufficiency in patients before operation for conal ventricular septal defect (Kaplan–Meier technique). Source: Reproduced by permission from Backer CL et al. J Thorac Cardiovasc Surg. 1991;102:288–296. Figure 16.19 Right atrial approach for ventricular septal defect (VSD) closure after aortobicaval cannulation and aortic cross‐clamp with cardioplegia. The tricuspid valvar leaflets are retracted to expose the VSD and the important surrounding structures. AV, atrioventricular. Source: Reproduced by permission from Mavroudis C et al., in Pediatric Cardiac Surgery, 3rd ed., Philadelphia, PA: Mosby; 2003, pp. 298–330. This surgical approach (Figure 16.19) [56, 93] is the most frequently used and is usually applicable to central or perimembranous defects, all those opening to the inlet of the right ventricle, most muscular defects, and the Gerbode types. Closure of muscular defects is usually first attempted through the right atrium. With some types, nonetheless, such as those opening at the right ventricular apex or the Swiss‐cheese variant, a limited right or left apical ventricular incision may be required. In addition to the general tenets regarding the cavitary incision and the conduct of extracorporeal circulation, many established techniques may be helpful in facilitating exposure with these different approaches during surgical VSD closure. The use of aortic cross‐clamping, cardioplegia, hypothermia, and a left ventricular vent is important not only to protect the myocardium and provide a relatively bloodless intracardiac surgical field, but also to relax the myocardium in order to optimize exposure. The view through the tricuspid valvar orifice may not be sufficient to effect complete and simultaneous exposure of all areas and the extent of the defect. When the heart is relaxed, changing the direction and tension of retractors will permit viewing the lesion one sector at a time. While others prefer a running suture technique to effect VSD closure, we prefer an interrupted suture technique to insure effective and complete VSD closure. It is usual to close the defect with 5‐0 double‐armed sutures, with buttressing pledgets, placed 2–3 mm away from its edge on the right ventricular side, permitting a wider contact area between the patch and the myocardium, helping to avoid injury to the conduction bundle that often runs near the crest of the defect, usually on the left ventricular side. Suture placement and patch positioning are illustrated in Figures 16.20–16.28. Frequently the defects in the perimembranous area may be covered by the septal leaflet of the tricuspid valve and may not be visualized immediately. This occurs particularly in those cases when the chordal attachments of the tricuspid valve to the edges of the defect are short and tight, or when there is formation of a pouch by redundant or accessory valvar tissue. In these instances, a clue to the location may be a depression in the tricuspid valve tissue being sucked into the defect by the action of the left ventricular vent. At times, the surgeon may be misled to believe that a small opening in the valvar tissue represents the total extent of the VSD (Figures 16.29 and 16.30) [33, 56]. In this circumstance, there may be a temptation to use simple direct closure with a suture, thus leaving the defect open behind the leaflet. This may result in residual shunting through another unrecognized open part of the defect. In order to avoid this pitfall, the hinge of the tricuspid valvar leaflets should be opened in a radial fashion (Figure 16.31) [33, 56, 93
CHAPTER 16
Ventricular Septal Defect
Definition and Prevalence
Historical Perspectives
Embryology and Pathologic Anatomy
Juxtapulmonary and doubly committed ventricular septal defect
Unifying phenotypic element is fibrous continuity between the leaflets of the pulmonary and aortic valves in its roof. The area of fibrous continuity, often seen as a fibrous remnant of the outlet septum, can be malaligned in cranial or caudal fashion relative to the muscular ventricular septum.
Central or perimembranous ventricular septal defect
Unifying phenotypic element is the border comprised by fibrous continuity between the leaflets of the aortic and tricuspid valves.
Central or perimembranous ventricular septal defect subtypes
Muscular ventricular septal defects
Unifying phenotypic element is that all such defects have exclusively muscular borders.
Muscular ventricular septal defects can occur geographically anywhere in the interventricular septum. When opening to the outlet of the right ventricle, they can be found with cranial or caudal malalignment of the muscular outlet septum.
Pathophysiology
Natural History
Diagnosis
Medical Management
Patient Selection
Patients with Large Ventricular Septal Defects
Patients with Small Defects
Patients with Defects Opening Directly beneath the Pulmonary Valve (Doubly Committed and Juxtaarterial Ventricular Septal Defects)
Surgical Considerations
Right Atrial Approach
Transatrial Closure of Perimembranous or Central Ventricular Septal Defect: Exposure by Tricuspid Valve Retraction
Transatrial Closure of Perimembranous or Central Ventricular Septal Defect: Exposure by Tricuspid Valve Incision
Stay updated, free articles. Join our Telegram channel
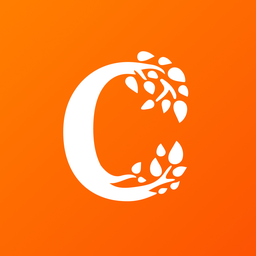
Full access? Get Clinical Tree
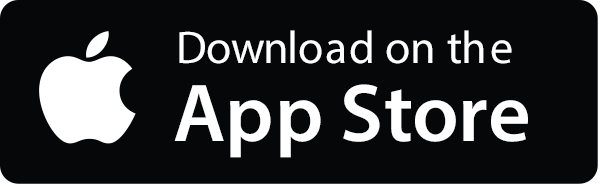
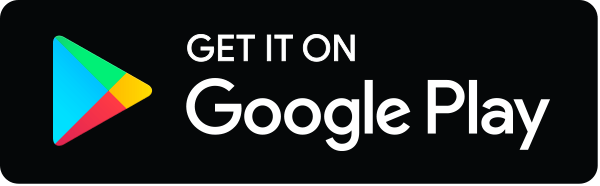