Venous Anatomy and Hemodynamics
Mark H. Meissner
The hemodynamics of the lower extremity venous system is in many respects more complicated and less well understood than it is for the arterial system. The central return of blood in the upright position depends on complex interactions between the heart, respiratory changes in venous pressure, the peripheral muscle pumps, and a multiply interconnected series of valved conduits. Failure of these components leads to a spectrum of chronic venous disorders ranging from uncomplicated telangiectasias and varicose veins to venous ulceration. The signs and symptoms may result from either primary degenerative causes or from secondary disorders, most commonly acute deep venous thrombosis (DVT). Regardless of etiology, the ultimate clinical and hemodynamic manifestations are similar. The more severe skin manifestations of chronic venous disease, termed chronic venous insufficiency, are specifically associated with ambulatory venous hypertension, or the failure to adequately reduce venous pressure with exercise.1
LOWER EXTREMITY VENOUS ANATOMY
Any description of lower extremity venous hemodynamics requires a clear understanding of venous anatomy. The nomenclature of the lower extremity veins was updated in 2002, clarifying many definitions and eliminating most eponyms.2 The venous system of the lower extremities includes the deep veins, which lie beneath the muscular fascia and drain the lower extremity muscles; the superficial veins, which are above the deep fascia and drain the cutaneous microcirculation; and the perforating veins that penetrate the muscular fascia and connect the superficial and deep veins. Communicating veins connect veins of the same anatomic type.2 However, it has been more recently emphasized that the lower extremity veins actually lie in three compartments—the deep veins in the compartment below the muscular fascia (N3), the great and small saphenous veins enveloped by the thin saphenous fascia (N2 compartment), and the saphenous tributaries within the epifascial (N1) compartment.3
The deep veins of the lower extremity follow the course of the associated arteries, with the number of valves increasing from proximal to distal. The muscular venous sinuses are the principal collecting system of the calf muscle pump. The gastrocnemius veins collect blood from the medial and lateral heads of the gastrocnemius muscle and drain into the popliteal vein, while the large soleus veins are of greatest numeric importance and usually drain into the posterior tibial or peroneal veins. These veins play an important role in the muscle pump function of the calf.
The superficial veins, which include the great saphenous vein and small saphenous vein, drain the skin and subcutaneous tissue. The principle veins of the superficial system of the medial thigh are the great saphenous vein and the anterior and posterior accessory great saphenous veins, all of which may be important sources of reflux. There are usually two main saphenous tributaries in the calf, an anterior branch and the posterior arch (Leonardo’s) vein, which begins behind the medial malleolus and joins the great saphenous vein just below the knee. The intersaphenous vein (vein of Giacomini) connects the small saphenous and great saphenous veins. The superficial venous system is completed by the lateral subdermic plexus formed by a network of small veins located laterally above and below the knee.4 Reflux in the lateral subdermic plexus is particularly associated with telangiectasias around the lateral knee.
Perforating veins may empty directly into the axial deep veins (direct perforators) or into the venous sinuses of the calf (indirect perforators). Although perforating veins are numerous and variable, four groups are of clinical significance— those of the foot, the medial calf, the lateral calf, and the thigh. Although net flow is from superficial to deep in the calf and thigh, the foot perforators are unique in directing flow toward the superficial veins.5,6 The medial calf perforators include the paratibial perforators joining the main great saphenous vein or its branches and the posterior tibial perforators that originate in the posterior arch vein. The perforators of the femoral canal connect the great saphenous vein with the distal femoral or proximal popliteal vein.
VENOUS HEMODYNAMICS
The lower extremity venous system functions both as a reservoir to facilitate cardiovascular homeostasis through volume shifts and to ensure the efficient return of blood to the heart. The elliptical cross-section of the lower extremity veins allows large volume shifts to be accommodated with minimal change in pressure over a range of 5 to 25 mm Hg. Veins are collapsible tubes and exhibit nonlinear volume-pressure relationships such that an increase in volume initially causes a “bending” of the wall associated with an increase in cross-sectional area without changes in pressure or perimeter (Fig. 6.1). Once a circular configuration is reached, the vein wall enters
a “stretching” phase, where further increases in volume are associated with increases in both perimeter and pressure.7 This arrangement allows large acute volume shifts to be accommodated by expansion or contraction of the venous reservoir.
a “stretching” phase, where further increases in volume are associated with increases in both perimeter and pressure.7 This arrangement allows large acute volume shifts to be accommodated by expansion or contraction of the venous reservoir.
Despite the importance of this reservoir capacity, the primary function of the venous circulation is to return blood to the heart. Lower extremity venous return is determined by the interaction of the dynamic pressure gradient between the lower extremities and the right atrium, respiratory-dependent changes in intra-abdominal and intrathoracic pressure (the respiratory pump), and the skeletal muscle pumps. In the resting supine position, the pressure is 12 to 18 mm Hg at the venous end of the capillary and falls steadily toward atrial pressures of 4 to 7 mm Hg.1 In the supine position, this dynamic pressure gradient is augmented by the effects of the respiratory pump. Although venous return is usually regarded as being augmented during inspiration, the situation is complex and depends on breathing mechanics.8 With diaphragmatic breathing, femoral venous return is abolished during inspiration and augmented during expiration (Fig. 6.2). This pattern is dictated by changes in intra-abdominal pressure rather than other factors such as constriction of the inferior vena cava at the diaphragmatic hiatus. In contrast, femoral venous blood flow is always positive during ribcage breathing, being slightly augmented during inspiration. The capacitance of the thigh veins appears to be the most important contributor to augmentation of venous flow induced by respiration.
In the upright position, the physiological effects of gravity and hydrostatic pressure oppose venous return. Resting venous pressure in the upright position rises by approximately 0.8 mm Hg per centimeter below the right atrium and reaches 80 to 100 mm Hg at the ankle, varying with height, weight, and calf muscle volume.9,10 At rest, arterial inflow is balanced by the action of the respiratory pump, and pressure in the deep and superficial veins is equal. However, as arterial inflow rises with activity, effective venous return requires the interaction of the peripheral venous pumps and competent venous valves.11,12,13,14,15 Despite the importance of the skeletal muscle pumps, there continues to be some respiratory modulation of venous return during exercise.8
The valves function to divide the hydrostatic column of blood into segments and to insure unidirectional, antegrade venous flow. The superficial, deep, and most perforating veins contain bicuspid valves consisting of folds of endothelium supported by a thin layer of connective tissue. Although often believed to be oriented parallel to the skin, the angle between the free edges of adjacent valves averages 84 degrees in the great saphenous vein and 88 degrees in the femoral vein.16 The offsetting of adjacent valves likely induces rotational momentum and helical flow in the veins, a strategy that limits flow instability and decreases energy dissipation elsewhere in the circulation. The valve cups lie within the valve sinus, which is always wider than the adjacent vein. The valve sinus enlarges and assumes a more spherical shape at the time of valve closure.17 Distension of the valve sinus separates the commissures, tightening the edge of the cusps and resulting in valve closure.
Within the deep venous system, there are an average of 5 venous valves between the inguinal ligament and popliteal fossa, although the number varies from 2 to 9.18 These valves are usually 3 to 5 cm apart and tend to be consistently positioned at major venous junctions.16 Their arrangement is
variable elsewhere, but in general, the inferior vena cava and common iliac veins have no valves; the external iliac and common femoral veins above the saphenofemoral junction have one valve at most; the femoral vein above the adductor canal has three or more valves; the distal femoral and popliteal veins have one or two valves; and the tibial/peroneal veins have numerous valves spaced at approximately 2 cm intervals.6,18 Although the muscle venous sinusoids are valveless, they frequently empty into profusely valved draining veins.18 Within the superficial venous system, a valve is present at the saphenofemoral junction in 94% to 100% of individuals19 and there are usually one or two subterminal valves within 2 cm of the terminal valve.4 The great saphenous vein usually has at least 6 valves, while the small saphenous vein has 7 to 10 closely spaced valves.6 Although the perforating veins of the calf generally direct flow from superficial to deep, most evidence suggest that only the larger perforating veins have valves.6,20
variable elsewhere, but in general, the inferior vena cava and common iliac veins have no valves; the external iliac and common femoral veins above the saphenofemoral junction have one valve at most; the femoral vein above the adductor canal has three or more valves; the distal femoral and popliteal veins have one or two valves; and the tibial/peroneal veins have numerous valves spaced at approximately 2 cm intervals.6,18 Although the muscle venous sinusoids are valveless, they frequently empty into profusely valved draining veins.18 Within the superficial venous system, a valve is present at the saphenofemoral junction in 94% to 100% of individuals19 and there are usually one or two subterminal valves within 2 cm of the terminal valve.4 The great saphenous vein usually has at least 6 valves, while the small saphenous vein has 7 to 10 closely spaced valves.6 Although the perforating veins of the calf generally direct flow from superficial to deep, most evidence suggest that only the larger perforating veins have valves.6,20
The valves of the lower extremity veins open and close at a cycle frequency of 34 to 36 per minute in the supine position and 19 to 20 per minute in the standing position.21 Knowledge regarding the mechanism of valve closure is largely derived from duplex observations of valves in response to nonphysiological provocative maneuvers such as Valsalva’s maneuver or distal calf compression. Under such conditions, valve closure is initiated by reversal of the normal, antegrade transvalvular pressure gradient during the relaxation or diastolic phase after calf muscle compression.22 As this pressure gradient reversal gradually increases, there is initially a short period of retrograde flow until the gradient becomes sufficient to cause valve closure. Rather than simple cessation of antegrade flow, valve closure under these artificial conditions requires retrograde flow at a velocity of at least 30 cm/s. Under such conditions, reverse flow duration is less than 0.5 seconds in 95% of normal valves.
Recent ultrasound observations under physiologic conditions, without augmentation or provocative maneuvers, suggest a different mechanism of valve closure.17,21 The valve cycle can be divided into four phases: opening, equilibrium, closing, and closed. At equilibrium, the valves are open but not apposed to the venous wall, causing a luminal narrowing of approximately 35% in comparison to the distal vein (Fig. 6.3A and B). This relative stenosis causes flow acceleration at the valve cusp with flow separation at the leading edge producing a vortex in the valve sinus. According to Bernoulli’s law, pressure decreases as velocity increases. Therefore, as luminal velocity increases at the end of the equilibrium phase, luminal pressure accordingly decreases, causing the valve to close when the vortex pressure behind the cusp exceeds the luminal pressure (Fig. 6.4). Among 12 normal subjects examined at rest in the upright position, flow reversal during valve closure occurred in only 1 subject. In this setting, cusp closure is initiated by an increase in forward flow velocity that is followed by a dilation of the valve sinus and subsequent valve closure. In distinct contrast to the mechanism of valve closure under the artificial conditions of a provocative maneuver, reverse flow is not necessary under normal physiologic conditions, and valve closure occurs despite ongoing antegrade flow.
Approximately 90% of the venous return in the lower extremities is via the deep veins through the action of the foot, calf, and thigh muscles pumps.23 The action of these valved pumps is dependent on normally functioning muscle groups, a full range of joint mobility, and the deep fascia of the leg which constrains the muscles during contraction and allows high pressures to be generated within the muscular compartments.24 Among these, the calf muscle pump is the most important as it is the most efficient, has the largest capacitance, and generates the highest pressures.5,13 The normal calf pump ejects over 40% to 60% of the venous volume with a single contraction.12,13,25 The thigh muscle pump, which has an ejection fraction of only 15%, is thought to be substantially less important, while compression of the plantar venous plexus likely functions to prime the calf muscle pump.15
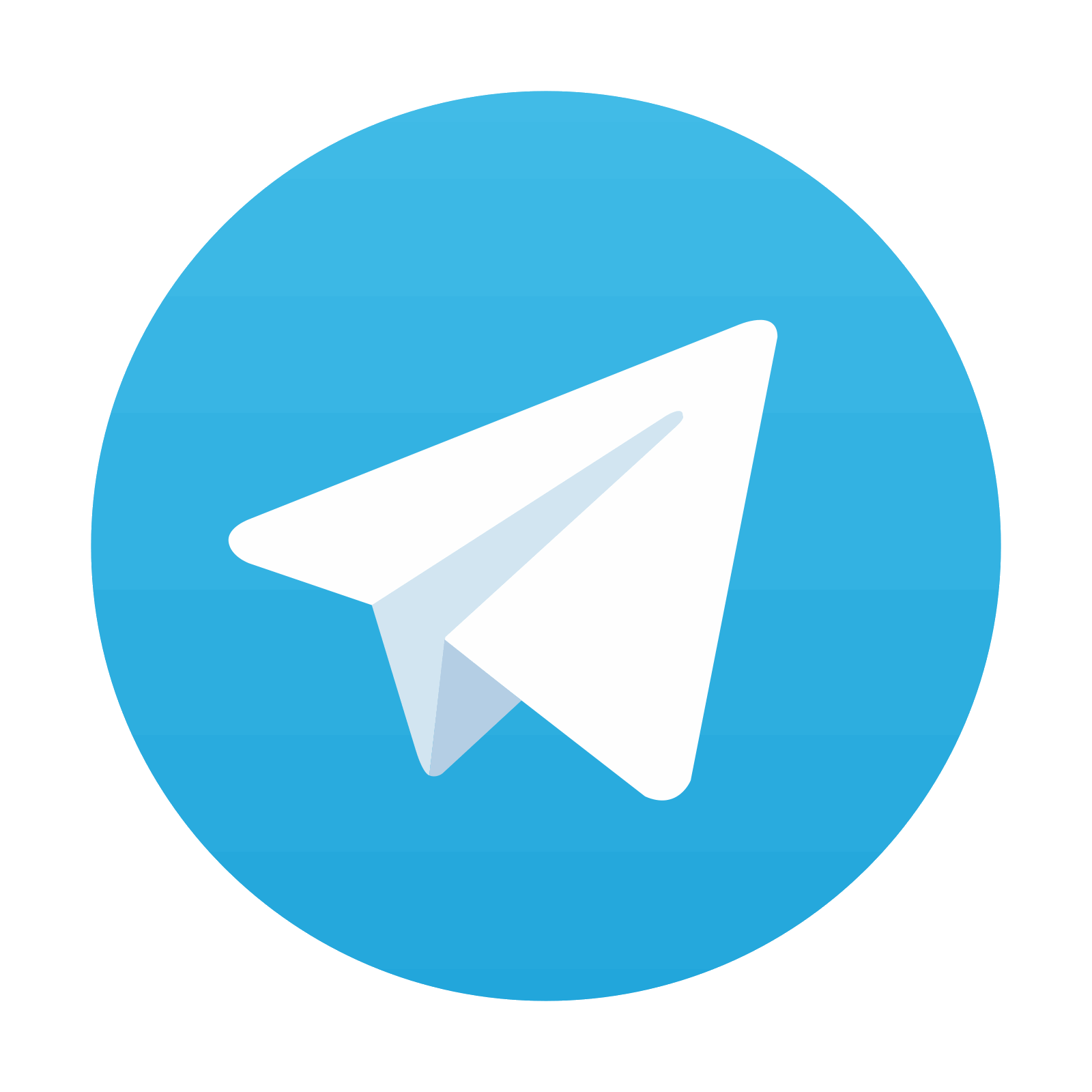
Stay updated, free articles. Join our Telegram channel
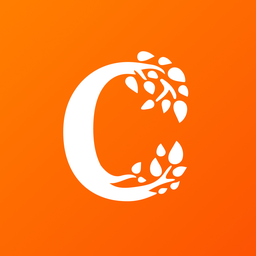
Full access? Get Clinical Tree
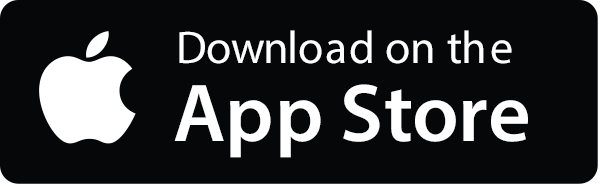
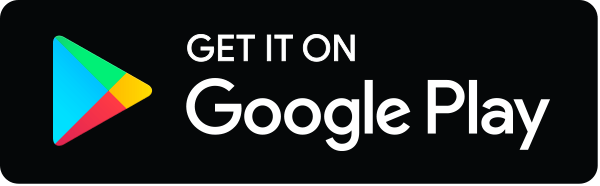