Abstract
This chapter describes some of the multiple molecular mechanisms that regulate the contraction of the vascular smooth muscle cell and thus the arterial vascular tone. This tone is central to the regulation of blood flow to tissue and thereby the distribution of cardiac output. In the newborn infant, the large distributary arteries take an active part in this. In the normal newborn infant, cardiac output is distributed equally to the upper and lower part of the body and the perfusion rate (in mL/100 g/min) is higher in liver and kidneys, as compared to the brain. Blood flow to the brain has been most actively studied, and autoregulation is already developed at the limit of viability, although autoregulation may be impaired and best be described as a degree of capacity rather than an on-off phenomenon. Vascular reactivity to P co 2 and to hypoxia is robust. The sympathetic nervous system plays a particular role in newborn infants and may be responsible for the decrease in blood flow to the cerebral hemispheres during the diving reflex as elicited by hypoxia.
Keywords
arterial blood pressure, arterial tone, autoregulation, diving reflex, newborn infant, P co 2 , sympathetic nervous system, vascular smooth muscle cell
- •
Multiple mechanisms operate to regulate blood flow to organs—these are also important in the newborn.
- •
Distribution of cardiac output is actively regulated.
- •
Cerebral autoregulation, that is, the ability to buffer the effects of blood pressure on cerebral blood flow, is developed even at the limits of viability.
- •
Cerebral autoregulation may best be described as a degree of capacity rather than an on-off phenomenon.
- •
A “diving” reflex-like response serves to prioritize vital organs during stress.
- •
The cerebral hemispheres may not be privileged, especially in the very preterm neonate.
In most organs, the principal role of perfusion is to provide substrates for cellular energy metabolism, with the final purpose of maintaining normal intracellular concentrations of the high-energy phosphate metabolites adenosine triphosphate (ATP) and phosphocreatine. The critical substrate is usually oxygen. Accordingly, organ blood flow is regulated by the energy demand of the given tissue. For instance, during maximal activation by seizures in the brain, cerebral blood flow (CBF) increases threefold, while in the muscle during maximal exercise, blood flow increases by up to eightfold. In addition, some organs, such as the brain, heart, and liver, have higher baseline oxygen and thus higher blood flow demand than others. Finally, in the kidney and skin, perfusion may be considerably above the metabolic needs to serve for glomerular filtration and thermoregulation, respectively. Indeed, during heating, skin blood flow may increase by as much as fourfold without any increase in energy demand.
In the developing organism, metabolic requirements are increased by as much as 40% due to the expenditures of growth. Since growth involves deposition of protein and fat, energy metabolism and, in particular, oxygen requirement are not increased as much as the requirements for protein and energy.
When blood flow is failing, there are several lines of defense mechanisms at the tissue level before the tissue is damaged. First, more oxygen is extracted from the blood. Normal oxygen extraction is about 30%, resulting in a venous oxygen saturation ( S v o 2 ) of 65% to 70%. Oxygen extraction can increase up to 50% to 60%, resulting in a S v o 2 of 40% to 50%, which corresponds to a venous (i.e., end-capillary) oxygen tension of 3 to 4 kPa. This is the critical value for oxygen tension for driving the diffusion of molecular oxygen from the capillary into the cell and to the mitochondria ( Fig. 2.1 ). Second, microvascular anatomy and the pathophysiology of the underlying disease process are both important for the final steps of oxygen delivery to tissue. When the cell senses oxygen insufficiency, its function is affected as growth stops, organ function fails, and, finally, cellular and thus organ survival are threatened ( Fig. 2.2 ). Ischemia is the term used for inadequate blood flow to maintain appropriate oxygen delivery and thus cellular function and integrity. Since there are several steps in the cellular reaction to oxygen insufficiency, more than one ischemic threshold may be defined. It is even possible that newborn infants can be, at least, partly protected against hypoxic-ischemic injury by mechanisms akin to hibernation by “hypoxic hypometabolism.”


The immature mammal is also able to “centralize” blood flow during periods of stress. This pattern of flow distribution is often called the “diving reflex,” since it is qualitatively similar to the adaptation of circulation in seals during diving, a process that allows sea mammals to stay under water for 20 minutes or more. Blood flow to the skin, muscle, kidneys, liver, and other nonvital organs is reduced to spare the oxygen reserve for the vital organs: the brain, heart, and adrenals. This reaction is relevant during birth with the limitations on placental oxygen transport imposed by uterine contractions and has been studied intensively in the fetal lamb. It has the potential of prolonging passive survival at a critical moment in the individual’s life. For comparison, the “fight-or-flight” response of the mature terrestrial mammal supports sustained maximal muscle work.
Physically, blood simply flows toward the point of lowest resistance. While flow velocities in the heart are high to allow the kinetic energy of the blood to thrust forward, flow velocity is minimal in the peripheral circulation. Organs are perfused simultaneously and the blood flow through a given tissue is the result of the pressure gradient between the arteries and the veins, the so-called perfusion pressure. Vascular resistance is composed of the limited diameter of blood vessels, particularly the smaller arteries and arterioles, and blood viscosity. The regulation of organ blood flow takes place by modifying arterial diameter, that is, by varying the tone of the smooth muscle cells of the arterial wall. Factors that influence vascular resistance are usually divided into four categories: blood pressure, chemical (P co 2 and P o 2 ), metabolic (functional activation), and neurogenic. Most studies of vascular resistance have been done on cerebral vessels. Therefore, the following account refers to cerebral vessels from mature animals, unless stated otherwise.
Regulation of Arterial Tone
The Role of Conduit Arteries in Regulating Vascular Resistance
It is usually assumed that the arteriole, the precapillary muscular artery with a diameter of 20 to 50 μm, is the primary determinant of vascular resistance, while the larger arteries are more or less considered passive conduits. However, this is not the case. For instance, in the adult cat the pressure in the small cerebral arteries (150 to 200 μm) is only 50% to 60% of the aortic pressure. Thus the reactivity of the entire muscular arterial tree is of relevance in regulating organ blood flow. The role of the prearteriolar vessels is likely even more important in the newborn than in the adult. First, the smaller body size translates to smaller conduit arteries. The resistance is proportional to length but is inversely proportional to the diameter to the power of 4. Therefore the conduit arteries of the newborn will make an even more important contribution to the vascular resistance. Second, conduit arteries, the largest arteries with an enhanced ability to stretch in response to pulsating flow, are very reactive in the newborn. The diameter of the carotid artery increases by 75% during acute asphyxia in term lambs, whereas the diameter of the descending aorta decreases by 15% when blood pressure drops. Thus, the former response must indicate active vasodilation. For comparison, flow-induced vasodilatation in the forearm in adults is only in the order of 5%. As resistance is proportional to the diameter to the power of 4, the findings in asphyxiated lambs indicate a roughly 90% reduction of the arterial component of the cerebrovascular resistance coupled with a near doubling of the arterial component of vascular resistance to the lower body. Incidentally, these observations also suggest that blood flow velocity, as recorded from conduit arteries by Doppler ultrasound, may be potentially misleading in the neonatal patient population.
Arterial Reaction to Pressure (Autoregulation)
Smooth muscle cells of the arterial wall constrict in response to increased intravascular pressure in the local arterial segment to a degree that more than compensates for the passive stretching of the vessel wall by the increased pressure. The net result is that arteries constrict when pressure increases and dilate when pressure drops. This phenomenon is called the autoregulation of blood flow ( Fig. 2.3 ). The response time in isolated, cannulated arterial segments is in the order of 10 seconds. The cellular mechanisms of this process are now better understood. Vessel wall constriction constitutes an intrinsic myogenic reflex and is independent of endothelial function. Rather, pressure induces an increase in the smooth muscle cell membrane potential, which regulates vascular smooth muscle cell activity through the action of voltage-gated calcium channels. Although the precise mechanism of the mechanochemical coupling is unknown, several plasma-membrane-bound receptors are involved, including G-protein-coupled receptors and a class of transient potential receptors. Furthermore, the calcium signal is modulated in many ways but a detailed discussion of this topic is beyond the scope of this chapter. Suffice to mention that phospholipases and activation of protein kinase C are involved, and, at least in the rat middle cerebral artery, the arachidonic acid metabolite 20-HETE has also been implicated. Furthermore, additional modulation of intracellular calcium concentration by alternative sources, such as the calcium-dependent K + channels, also exists. Local variations in the expression of these receptors and channels may, at least in part, explain the difference in responses to blood pressure in different vascular beds and the unique blood flow distribution between vital and nonvital organs.

Interaction of Autoregulation and Hypoxic Vasodilatation
As described earlier, arterial smooth muscle tone is affected by a number of factors, all contributing to determine the incident level of vascular resistance. Among the vasodilators, hypoxia is one of the more potent and physiologically relevant factors. Vascular reactivity to O 2 depends, in part, on intact endothelial function ensuring appropriate local nitric oxide (NO) production. Hypoxia also induces tissue lactic acidosis. The decreased pH constitutes a point of interaction between O 2 and CO 2 reactivity (see later). In addition, hypoxia decreases smooth muscle membrane potential by the direct and selective opening of both the calcium-activated and ATP-sensitive K + channels in the cell membrane. In the immature brain, adenosine is also an important regulator of the vascular response to hypoxia. The membrane potential response to hypoxia is independent of the existing intravascular pressure. However, at lower pressures, the decrease in membrane potential only leads to minimal further arterial dilation because, at low vascular tone, the membrane potential-muscular tone relationship is outside the steep part of the slope ( Fig. 2.4 ). In other words, at low perfusion pressures, the dilator pathway has already been near maximally activated. Therefore, in a hypotensive neonate, a superimposed hypoxic event cannot be appropriately compensated due to the low perfusion pressure. The end result is tissue hypoxia-ischemia with the potential of causing irreversible damage to organs, especially to the brain. This is a clinically highly relevant point when providing care for the hypotensive and hypoxic neonate.

Interaction of Autoregulation and P co 2
Arteries and arterioles constrict with hypocapnia and dilate with hypercapnia. The principal part of this reaction is mediated through changes in pH, that is, H + concentration. Perivascular pH has a direct effect on the membrane potential of arterial smooth muscle cells since, mostly via activation of both the ATP-sensitive and calcium-activated K + channels, extracellular H + concentration is one of the main determinants of the potassium conductance of the plasma membrane in arterial smooth muscle cells regulating the outward K + current. Therefore, when the pH decreases, the K + outflow from the vascular smooth muscle cell increases, resulting in hyperpolarization of the cell membrane and, via the closure of voltage-gated Ca ++ channels, it causes vasodilatation. Furthermore, increased extracellular and, to a lesser degree, intracellular H + concentrations reduce the conductance of the voltage-dependent calcium channels, further enhancing vascular smooth muscle cell relaxation.
Hypercapnic vasodilatation is reduced by up to 50% when NO synthase (NOS) activity is blocked in the brain of the adult rat. The hypercapnic response is restituted by the addition of an NO donor. This finding suggests that unhindered local NO production is necessary for the pH to exert its vasoregulatory effects. Indeed, it has been suggested that, although the calcium-activated and ATP-sensitive K + channels play the primary role in the vascular response to changes in P co 2 , the function of these channels is regulated by local NO production.
The role of prostanoids in mediating the vascular response to P co 2 is less clear. The fact that indomethacin abolishes the normal cerebral (or other organ) blood flow–CO 2 response in preterm infants is likely a direct effect of the drug independent of its inhibitory action on prostanoid synthesis. This notion is supported by the finding that ibuprofen is devoid of such effects on the organ blood flow–CO 2 response.
Interaction of Autoregulation and Functional Activation (Metabolic Blood Flow Control)
Several mechanisms operate to match local blood flow to metabolic requirements, including changes in pH, local production of adenosine, ATP and NO, and local neural mechanisms. It appears that, in the muscle, there is not a single factor dominating, since the robust and very fast coupling of activity and blood flow is almost unaffected by blocking any of these mechanisms one by one. In the brain, astrocytes may be the central sites of regulation of this response in the neurovascular unit via their perivascular end-feet and by using many of the aforementioned cellular mechanisms, such as changes in K + ion flux and the local production of prostanoids, ATP, and adenosine. Among these cellular regulators, adenosine has been proposed to play a principal role. Adenosine also works by regulating the activity of the calcium-activated and ATP-sensitive K + channels.
Flow-Mediated Vasodilatation
Endothelial cells sense flow by shear stress and produce NO in reaction to high shear stress at high flow velocities. NO diffuses freely and reaches the smooth muscle cell underneath the endothelium. NO acts on smooth muscle K + channels using cyclic guanosine monophosphate (GMP) as the secondary messenger and then a series of intermediate steps. Since NO is a vasodilator, the basic arterial reflex to high flow is vasodilation. Thus when a tissue is activated (e.g., a muscle contracts), the local vessels first dilate, as directed by the mechanisms of the metabolic flow control described earlier, and blood flow increases. This initial increase in blood flow is then sensed in the conduit arteries through the shear stress-induced increase in local NO production, and vascular resistance is further reduced allowing flow to increase yet again. The action remains local as the generated NO diffusing into the bloodstream is largely inactivated by hemoglobin.
Sympathetic Nervous System
Epinephrine in the blood originates from the adrenal glands, whereas norepinephrine is produced by sympathetic nerve endings and extraadrenal chromaffin tissue. Sympathetic nerves are present in nearly all vessels, located in the adventitia and mixed in with the smooth muscle cells. Adrenoreceptors are widely distributed in the cardiovascular system located in the smooth muscle and endothelial cell membranes. Several different adrenoreceptors exist; alpha-1 receptors with at least three subtypes are present primarily in the arteries and the myocardium, while alpha-2, beta-1, and beta-2 receptors are expressed in all types of vessels and the myocardium. In the arteries and veins, alpha-receptor stimulation causes vasoconstriction, and beta-receptor stimulation results in vasodilatation. Both alpha- and beta-adrenoreceptors are frequently expressed in the membrane of the same cell. Therefore, the response of the given cell to epinephrine or norepinephrine depends on the relative abundance of the receptor types expressed. Of clinical importance is the regulation of the expression of the cardiovascular adrenergic receptors by corticosteroids, the high incidence of relative adrenal insufficiency in preterm neonates and critically ill term infants (see Chapter 30 ), the role of glucocorticoids and mineralocorticoids in maintaining the sensitivity of the cardiovascular system to endogenous and exogenous catecholamines, and the downregulation of the cardiovascular adrenergic receptors in response to the increased release of endogenous catecholamines or the administration of exogenous catecholamines in critical illness. Typically, arteries and arterioles of the skin, gut, and muscle constrict in response to increases in endogenous catecholamine production, whereas those of the heart and brain either do not constrict or even dilate (see later). The response also depends on the resting tone of the given vessel. Furthermore, the sensitivity of a vessel to circulating norepinephrine may be less than the sensitivity to norepinephrine produced by increased sympathetic nerve activity, since alpha-1 receptors may be particularly abundant in the membrane regions close to where the nerve secretes the transmitter. The signaling pathways of the adrenoreceptors are complex and dependent on the receptor subtype. Activation of alpha-adrenoreceptors generally results in vasoconstriction mediated by increased release of calcium from intracellular stores as a first step, while beta-receptor-induced vasodilation is mediated by increased cyclic adenosine monophosphate (AMP) generation. However, the system is far more complex and, among other mechanisms, receptor activation-associated changes in K + conductance and local NO synthesis are also involved. Finally, the sympathetic nervous system is activated during hypoxia, hypotension, or hypovolemia via the stimulation of different chemoreceptors and baroreceptors in the vessel walls and the vasomotor centers in the medulla. Activation of the sympathetic nervous system plays a central role in the cardiovascular response to stress and it is the mainstay of the diving reflex response during hypoxia-ischemia.
Humoral Factors in General Circulation
A large number of endogenous vasoactive factors, other than those mentioned earlier, also play a role in the extremely complex process of organ blood flow regulation, such as angiotensin II, arginine-vasopressin, vasointestinal peptide, neuropeptide gamma, and endothelin-1. However, none of these vasoactive factors has been shown to have a significant importance in isolation under normal conditions except for the role of angiotensin II in regulating renal microhemodynamics.
In conclusion, a great many factors have an input and interact to define the degree of contraction of the vascular smooth muscle cells and, hence, regulate arterial and arteriolar tone (see Fig. 2.4 ). Although many details are unknown, especially in the developing immature animal or human, the final common pathway appears to involve the smooth-cell membrane potential, cytoplasmic calcium concentration, and the calcium/calmodulin myosin light chain kinase-mediated phosphorylation of the regulatory light chains of myosin resulting in the interaction of actin and myosin ( Fig. 2.5 ). However, the complexity of the known factors and their interplay as well as the differences in the response among the different organs are overwhelming, and no simple or unifying principle of vascular tone regulation has gained a foothold. Indeed, the complexity predicts that vascular tone and reactivity in a particular arterial segment in a particular tissue may differ markedly from that in other segments or other tissues. Unfortunately, the insights are as yet insufficient to allow any quantitative predictions for different organs or vascular tree segments.

Blood Flow to the Brain
Brain injury is common in newborn infants. It can occur rapidly, is frequently irreversible, and rarely, in itself, prevents survival. Injury to no other organs in the neonatal period has the same clinical importance, as the other organs have a better capacity to recover even from severe hypoxic-ischemic damage. Disturbances in blood flow and inflammation have been proposed as the major factors in the development of neonatal brain injury.
Autoregulation of Cerebral Blood Flow in the Immature Brain
Pressure-flow autoregulation has been widely investigated in the immature cerebral vasculature since the original observation of direct proportionality of CBF to systolic blood pressure in a group of neonates during stabilization after birth.
An adequate autoregulatory plateau, shifted to the left to match the lower perinatal blood pressure, has been demonstrated in several animal species shortly after birth, including dogs, lambs, and rats. In fetal lambs, autoregulation is not present at 0.6 weeks’ gestation, but is functional at 0.9 weeks’ gestation. The lower threshold of the autoregulation is developmentally regulated and it is closer to the normal resting systemic mean blood pressure at 0.75 gestation compared with 0.9 gestation. Thus, in the more immature subject there is less vasodilator reserve, which limits the effectiveness of CBF autoregulation at the earlier stages of development. In newborn lambs, autoregulation could be completely abolished for 4 to 7 hours by 20 minutes of hypoxemia with arterial oxygen saturations about 50%.
Observational studies of global CBF in stable neonates without evidence of major brain injury suggest that autoregulation is intact. In contrast, evidence of absent autoregulation has been found under pathologic conditions, such as following severe birth asphyxia in term infants, and in preterm infants in association with brain injury or death.
Imaging of CBF during arterial hypotension suggested that CBF to the periventricular white matter may be selectively reduced at blood pressures less than 30 mm Hg, supporting the notion that the periventricular white matter is a “watershed area.” Another study also found some evidence for the lower threshold of the autoregulatory curve for the global CBF around 29 mm Hg.
In conclusion, the lower threshold for CBF autoregulation may be around 30 mm Hg or somewhat below, and autoregulation can be assumed to operate in most newborn infants, even the most immature. When blood pressure falls below the threshold, CBF will fall proportionally more due to the elastic reduction in vascular diameter. However, significant blood flow is believed to be present until systemic mean blood pressure is less than 20 mm Hg, and it may in general be more appropriate to consider arterial blood pressure-CBF reactivity (i.e., autoregulation) as a degree of capacity, rather than an on-off phenomenon. Finally, while poor clinical outcomes may be predicted by impaired autoregulation, it remains to be demonstrated that it has clinical value to estimate the state of the autoregulation. One way could be to determine the level of arterial blood pressure, where the pressure-flow reactivity is lowest—the optimal blood pressure—and then to treat arterial hypotension using this individualized target.
Effect of Carbon Dioxide on Cerebral Blood Flow
Changes in carbon dioxide tension (P co 2 ) have more pronounced effects on CBF than on blood flow in other organs due to the presence of the blood-brain barrier. The blood-brain barrier is an endothelium with tight junctions, which does not allow HCO 3 − to pass through readily. The restricted diffusion of HCO 3 − means that hypercapnia and hypocapnia decreases and increases, respectively, the pH in the perivascular space in the brain more readily than in blood where buffering is more effective due to the presence of hemoglobin. This response to a change in P co 2 continues until HCO 3 − equilibrates over the course of hours.
In normocapnic adults, small acute changes in arterial P co 2 (Pa co 2 ) result in a change in CBF by 30% per kPa (4% per mm Hg Pa co 2 ). Similar reactivity has been demonstrated in the normal human neonate by venous occlusion plethysmography and in stable preterm ventilated infants without major germinal layer hemorrhage by using the l33 Xe clearance technique. However, Pa co 2 reactivity is less than 30% per kPa during the first 24 hours.
Contrary to the vasodilation induced by increases in the P co 2 , a hyperventilation-related decrease in Pa co 2 causes hypocapnic cerebral vasoconstriction and has been found to be associated with brain injury in preterm but not in term infants or adults. It is an open question whether hypocapnia alone can cause ischemia, or if it works in combination with other factors, such as hypoxemia, hypoglycemia, the presence of high levels of cytokines, sympathetic activation, or seizures.
Metabolic Control of Blood Flow to the Brain
CBF in term infants, estimated by venous occlusion plethysmography, is greater during active sleep than during quiet sleep and, in preterm infants of 32 to 35 weeks’ postmenstrual age, in the wake state compared with sleep. Thus there is flow-metabolism coupling even before term gestation in the brain. This finding is further supported by the documented increase in CBF seen during seizure activity and by the strong relation between CBF and blood hemoglobin concentration.
The cerebrovascular response to functional activation by sensory stimulation was found to be variable by initial studies using magnetic resonance imaging and near-infrared spectroscopy (NIRS). More recently, a clear coupling between increases in local metabolic rate and blood flow has been demonstrated in preterm infants and also between burst activity and cerebral oxygenation in term infants with neonatal encephalopathy.
Cerebrovenous oxygen saturation was entirely normal (64% ± 5%) as estimated by NIRS and jugular occlusion technique in 11 healthy, term infants 3 days after birth. In a simple way, this finding indicates that there is a balance between blood flow and cerebral oxygen consumption in the newborn.
Adrenergic Mechanisms Affecting Cerebral Blood Flow
Based on findings in animal studies, the sympathetic system appears to play a greater role affecting CBF and its autoregulation in the perinatal period than it does later in life. This finding has been attributed to the relative immaturity of the NO-induced vasodilatory mechanisms during early development. The adrenergic effect, at least in part, results in enhanced constriction of conduit arteries.
A rare study of human neonatal arteries in vitro (obtained postmortem from preterm neonates of 23 to 34 weeks’ gestation) showed basal tone and a pressure-diameter relation quite similar to those seen in the adult pial arteries. However, the neonatal arteries were significantly more sensitive to exogenous norepinephrine and electrical field activation of adventitial sympathetic nerve fibers, and had a much higher sympathetic nerve density compared with those in the adult pial arteries.
Effect of Medications on Cerebral Blood Flow
Indomethacin reduces CBF in experimental animals, adults, and preterm neonates. The concern has been whether it reduces CBF to ischemic levels resulting in injury in the developing brain. Interestingly, although indomethacin decreases the incidence of severe peri/intraventricular hemorrhage, this early effect does not seem to translate to better long-term neurodevelopmental outcomes. Contrary to indomethacin, ibuprofen does not have significant cerebrovascular effects. However, it is not known whether the long-term neurodevelopmental outcome in neonates exposed to ibuprofen is better than in those having been treated with indomethacin.
Among the methylxanthines, aminophylline reduces CBF and Pa co 2 in experimental animals, adults, and preterm infants, but caffeine has less of an effect on CBF. Methylxanthines are potent adenosine receptor antagonists. However, it is not entirely clear whether the reduction of CBF is the direct effect of methylxanthines on the adenosine receptors, a result of the associated decrease in Pa co 2 , or a combination.
Dopamine does not appear to have a selective (dilatory) effect on brain vasculature and its administration has been found to be associated with impaired autoregulation. Individual studies have varied regarding its effect on CBF, but using a meta-analytic approach, dopamine administration results in increases in CBF in hypotensive neonates.
Ischemic Thresholds in the Brain
In the newborn puppy, S v o 2 may decrease from 75% to 40% without provoking significant lactate production. The exact minimum value of “normal” S v o 2 depends on, among other things, the oxygen dissociation curve. Therefore, it may be affected by changes in pH and the proportion of fetal hemoglobin present in the blood.
In the cerebral cortex of the adult baboon and man, the threshold of blood flow sufficient to maintain tissue integrity depends on the duration of the low flow. For instance, if the low flow lasts for a few hours, the limit of minimal CBF to maintain tissue integrity is around 10 mL/100 g/min. In acute localized brain ischemia, blood flow may remain sufficient to maintain structural integrity but fail to sustain functional (electrical) activity, a phenomenon called “border zone” or “penumbra.” Indeed, in progressing ischemia, electrical failure is a warning for the impending development of permanent tissue injury. In the adult human brain cortex, electrical function ceases at about 20 mL/100 g/min of blood flow, while in the subcortical gray matter and brainstem of the adult baboon, the blood flow threshold is around 10 to 15 mL/100 g/min.
In normal newborn infants at rest the global CBF is 15 to 20 mL/100 g/min (see Chapter 16 ). The ischaemic threshold values of CBF for neonates are not known. However, in view of the low resting levels of CBF and the comparatively longer survival in total ischemia or anoxia, neonatal CBF thresholds are likely to be considerably less than 10 mL/100 g/min. Indeed, in ventilated preterm infants visual evoked responses were unaffected at global CBF levels below 10 mL/100 g/min corresponding to a cerebral oxygen delivery of 50 μmol/100 g/min.
Low CBF and cerebral oxygen delivery carry a risk of later death, cerebral atrophy, or neurodevelopmental deficit, but it is unclear whether treatment aimed at increasing CBF (or its surrogate, cerebral oxygenation) can improve the outcome.
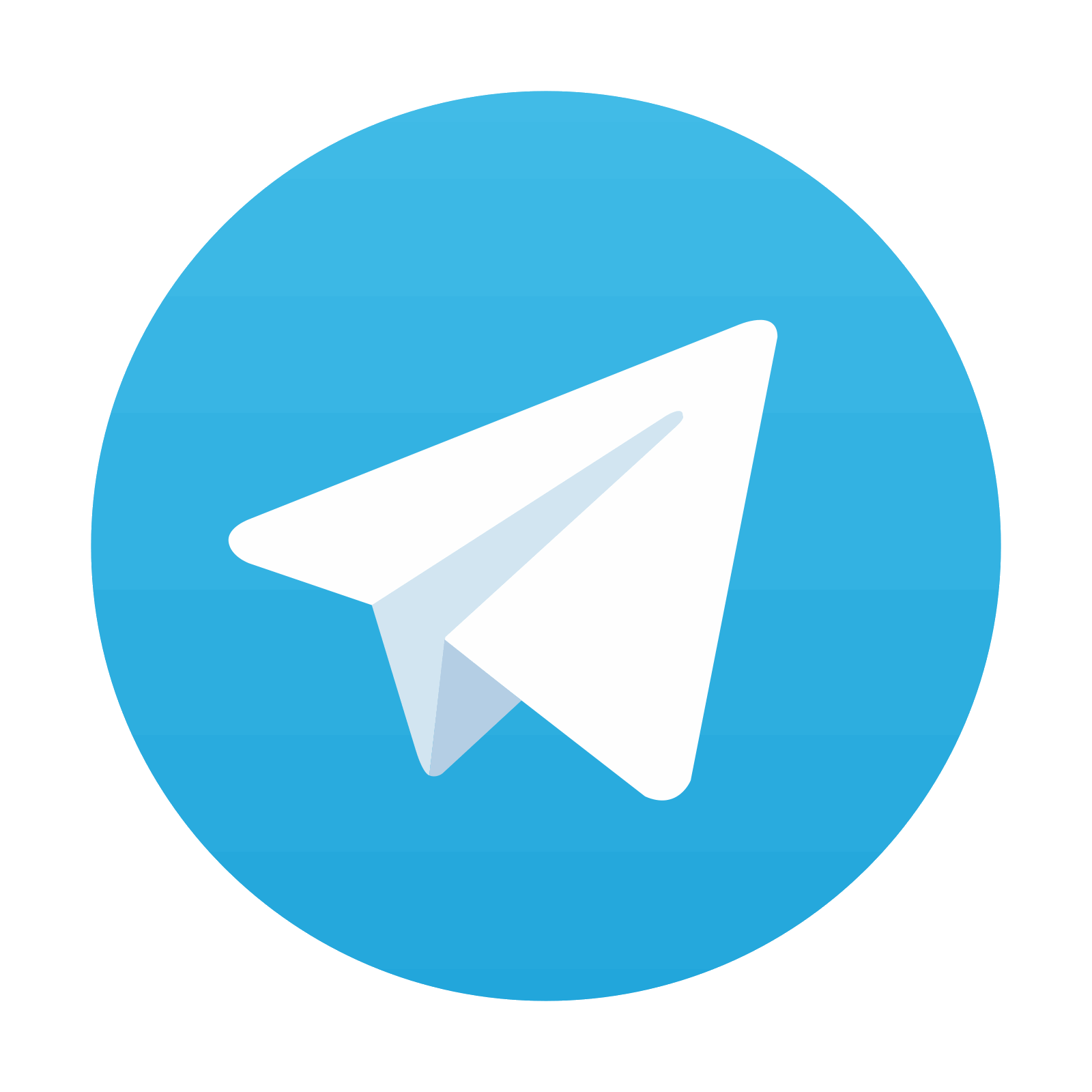
Stay updated, free articles. Join our Telegram channel
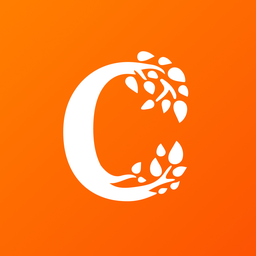
Full access? Get Clinical Tree
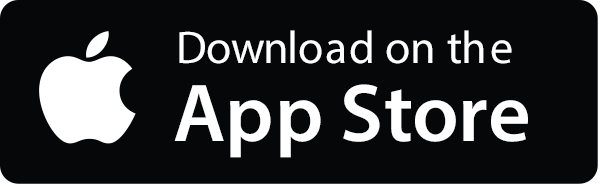
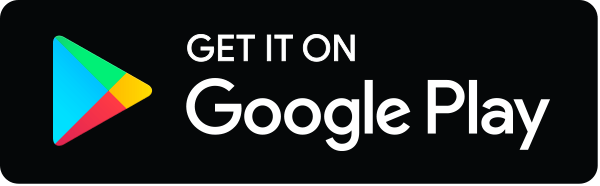