Chapter 16 Vascular Grafts
Characteristics and Rational Selection
Alexis Carrel, the father of vascular surgery, was the first to describe the utility and shortfalls of autogenous and synthetic grafts. The main limitation identified by him was the lack of durability for small-diameter synthetic grafts.1,2 Kunlin first used the saphenous vein for arterial bypass,3 but it took time to implement the use of vein as a preferred conduit for arterial grafting.4 The first clinical successes of synthetic vascular grafts were reported in the 1950s when Voorhees and colleagues developed the first fabric graft from Vinyon N, a material commonly used in parachutes5; several ethylene-based synthetic grafts followed. To date, large vessel grafts have demonstrated excellent results, whereas small-diameter grafts have performed poorly.
Cardiovascular disease has become more prevalent, and it is the leading cause of death in the world and the second leading cause of death for all Americans. Despite improvements in the medical therapy of cardiovascular disease, the number of vascular interventions (combining bypass grafting and angioplasty ± stenting) has at least doubled in recent years.6 Coupling this finding with an increasing lifespan, the population that will benefit from vascular surgery is expected to continue to increase. With more than 1.4 million bypass operations annually in the United States, finding suitable autogenous veins and arteries for conduit in patients with a lifespan of more than 2 years is critical to optimal therapy in preventing limb loss and delaying mortality.7 When suitable autogenous conduits are not available, synthetic grafts are useful, particularly for bypassing proximal disease.
Normal and Pathologic Composition of the Vessel Wall
The arterial intima (tunica intima) is composed of a relatively quiescent endothelial cell (EC) monolayer and its surrounding basement membrane proteins (e.g., type IV collagen, perlecan). Together with the underlying internal elastic lamina, the intima maintains smooth muscle cells (SMCs) in their contractile state and inhibits pathologic SMC activity. Deep to the intima and separated by the internal elastic lamina is the medial layer (tunica media). It is the thickest arterial layer and in non-pathologic states it is composed of SMCs and many extracellular matrix (ECM) proteins (e.g., elastin and the fibrillar collagens such as type I collagen). Medial SMCs here respond to intimal cues to dilate or contract the vessel. The medial layer in veins is difficult to define because it lacks the internal elastic lamina, but after exposure to arterial flow via vein graft bypass, they develop a defined media over time that has similarities to that of native artery.8 The external elastic lamina defines the abluminal edge of the media The vaso vasorum, which supplies most of the outer two thirds of the vessel wall’s metabolic needs, is prominent in the outer adventitial vessel layer (tunica adventitia). The adventitia is composed of loosely arranged connective tissue and fibroblasts, and it could have an important role in the progression of restenosis and late interventional failure after angioplasty owing to vessel remodeling, because adventitial delivery of therapeutic treatments has reduced these complications.9
Current Status of Vascular Conduits
The Artery
Few autogenous arteries are available for use as a conduit because of the lack of redundant arterial perfusion and available lengths of medium-sized arteries. However the patency rates of inferior mammary artery conduits in coronary artery bypass grafting is outstanding compared with vein grafts.10 The benefit is less clear when arterial harvesting is required, such as radial artery grafting.11 There is also some support for this benefit in patients with challenging distal bypasses.12 The theoretic advantage is that arteries do not need to adapt to arterial flow, and thus are relatively protected from negative remodeling. However, all bypass targets are not identical, and graft remodeling is also determined in part by the perfusion bed (myocardium versus skeletal muscle).
The Vein
Superficial and deep veins can be used as conduits for arterial bypass. Their benefit over synthetic grafts for small diameter graft bypasses is well accepted.13 The greater saphenous vein is the most commonly used conduit for coronary and infrainguinal bypass grafting. It is duplicated in approximately 8% of persons,14 and its course has been well described.15 The lesser saphenous vein is not easily accessible in the supine position, but it can be useful as a conduit when working posteriorly or through a separate harvest incision.16–18 More commonly in our practice, bilateral cephalic veins, which are approximately 50 cm, can be sewn together as a composite conduit for distal bypasses.19,20
Once implanted, veins undergo arterialization in which they develop a muscular media and elastic lamina. Adaption can be both positive and negative; therefore it is not surprising that a number of vein grafts fail or require adjunctive procedures to maintain patency (Table 16-1). The modes of failure include myointimal hyperplasia (frequently at anastomotic or valve sites or at sites of intraluminal manipulation) and progression of atherosclerosis in the inflow or outflow arteries, or both, or in the vein graft itself. Limiting trauma at time of vein harvest,21 proper perioperative medication (antiplatelet agents22 and a statin23,24), proper ultrasound surveillance protocols25 are critical to optimizing patency and clinical benefit of vein grafts. The patency benefit of anticoagulation of vein grafts is modest,26,27 and the risks of bleeding are generally thought to outweigh these small benefits to vein graft patency.24
TABLE 16-1 Proper Handling of Vein Grafts
Limiting Injury | Surveillance and Secondary Patency |
---|---|
Cryopreserved Vessels
Cryopreserved allografts provide an alternative to either femoral vein harvest or to antibiotic-soaked Dacron with omental flap coverage for infected aortoiliac reconstruction.32 However, their poor primary patency rates for infrainguinal bypass,33–35 even with immunosuppressive therapy,36 limit this application to patients with infection and without leg or arm vein.
Synthetic Grafts
The idea of a nonreactive vascular conduit as an ideal graft is no longer accepted and is likely impossible to create; therefore current initiatives emphasize incorporation into the surrounding tissues. These approaches include the development of nonautologous biologics, synthetic grafts that promote or support tissue ingrowth, and tissue engineered vascular grafts that attempt to mimic certain functional properties of the blood vessel, such as compliance and desirable cell phenotypic characteristics. Readily available large-diameter grafts, such as those used for aortic reconstruction, have superb 5-year patency rates.37 Conversely, when synthetic grafts are used in the infrapopliteal region, the results are poor, with 1- and 3-year patency rates of 43% and 30%, respectively.13
Conduit Patency and Failure
The benefits of vein grafts versus synthetic grafts is well established,13,38 but recently the PREVENT III and IV trials have demonstrated that even vein grafts have durability limitations for both infrainguinal and coronary revascularizations.39,40 As described, primary patency rates are strikingly similar to those reported more than 30 years ago.13 However, secondary patency rates are better, emphasizing the importance of vein graft surveillance and prophylactic reinterventions.
Pathophysiologically, denuded intima of a vein graft or exposed luminal area of a synthetic graft may lead to thrombosis via platelet deposition and activation of the coagulation cascade, which over time promotes pathologic SMC migration, proliferation, and ECM deposition, leading to IH. IH in turn narrows the vessel lumen (restenosis), decreasing blood flow to the point that it can promote local thrombosis or lead to symptomatic ischemia in the relevant distal end organs, such as the brain (cardiovascular accidents), heart (myocardial infarctions), and extremities (critical limb ischemia; Figure 16-1).
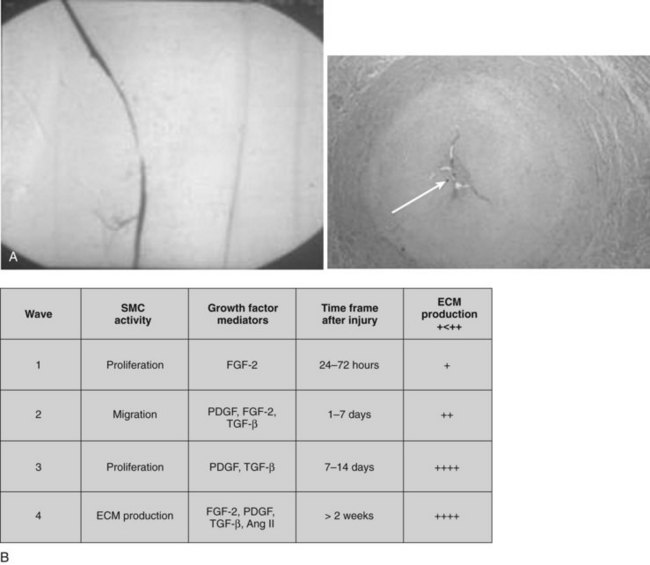
FIGURE 16-1 Myointimal hyperplasia: arteriographic and histologic findings.
(Reprinted with permission from Elsevier. Brewster LP, Brey EM, Greisler HG et al: Advanced Drug Delivery Reviews, 58 (9) 604–629, 2006.)
Cellular and Molecular Mediators of Graft Outcome
A confluent quiescent endothelial lining of injured vessels and synthetic grafts is desirable for clinical applications.41 Re-endothelialization of injured vessels or endothelialization of synthetic grafts can occur through transanastomotic endothelial cell migration (usually limited in humans to 1 to 2 cm), transmural tissue ingrowth accompanied by microvessels from the perigraft tissue, and from deposition of circulating endothelial cells, endothelial progenitor cells (EPCs), or stem cells. Homing can be promoted through affixation of EC attractant antibodies to the grafts in a fashion similar to that used in coronary artery stents.42
However, ECs growing on prosthetic graft surfaces are not necessarily the same as their normal quiescent counterparts in uninjured vessels. These ECs are often “activated,” secreting bioactive substances (e.g., platelet-derived growth factor [PDGF]) that actually promote thrombogenesis and changes in SMCs to a synthetic phenotype. This has been seen in the perianastomotic region, which is the most frequent site of interventional failure after operation.43 SMCs found within the myointima of prosthetic grafts are also functionally altered. They produce significantly higher amounts of PDGF, as well as various extracellular matrix proteins, than those of the adjacent vessel, which contribute to the development of intimal hyperplasia along with the body’s inflammatory reactions to prosthetic material.44
A variety of cytokines are released from inflammatory cells activated by vascular grafts. Cultured monocytes and macrophages incubated with Dacron and expanded polytetrafluoroethylene (ePTFE) have been demonstrated to produce different amounts of IL-1β, IL-6, and TNF-α that are biomaterial specific.45 Because the inflammatory reaction elicits a cascade of growth processes, it has been proposed that approaches attenuating the initial inflammatory reaction may improve long-term graft patency. Alternatively, directing the inflammatory response to promote favorable cellular and protein responses may promote intimal generation and tissue incorporation.
Creation of a More Hospitable Blood Biomaterial Interface
The long-term patency of vascular grafts depends upon the intrinsic properties of the graft itself and the hemodynamic environment in which the graft is placed, as well as patient variables (e.g., diabetes, renal failure, nutritional status), and may or may not be improved by prior or concomitant interventions such as proximal or distal angioplasty. It is now clear that tissue incorporation is important for graft function; therefore grafts that have this ability are considered desirable for medium- and small-caliber vessel replacement. The compliance mismatch between arteries and grafts causes flow disruption in vivo, which could contribute to anastomotic pseudointimal hyperplasia.46 For this reason, a number of surgeons have suggested interposing a segment of vein between the synthetic graft and artery, creating a composite graft at the distal anastomosis. This has also led some investigators to design more compliant grafts using more flexible materials or changing the parameters of graft construction to improve graft compliance. Although animal experiments have suggested concept validity, the clinical benefit of this approach remains controversial. Many factors may contribute to this confusion, including longitudinal variability in the diameter and compliance of the arterial tree and the effect of activated endothelium on intimal hyperplasia. Furthermore, there is a robust fibrotic response after implantation that leads compliant grafts to become more noncompliant after implantation; therefore, even if a compliance match were attained initially, this benefit would unlikely persist. Synthesis of a conduit designed to match the compliance of the arterial wall is simplistic, as the compliance of the diseased arteries to which the grafts would be anastomosed is widely variable. Furthermore, in the para-anastomotic region there are dynamic changes in compliance that vary over time. First a para-anastomotic hypercompliant zone exhibits a 50% gain in compliance, then later its compliance is lessened 60% from baseline.47 It is likely that this bimodal adaptation limits the practical value of graft designs focused on initial mismatch. Addressing later mismatch may be more valuable, but synthetic grafts are intrinsically limited in this ability to adapt over time.
Anticoagulant Affixation
Early platelet deposition on vascular grafts is mediated by von Willebrand factor and platelet membrane glycoproteins. After adherence to a graft, platelets degranulate, releasing many bioactive substances, including serotonin, epinephrine, ADP, and thromboxane A2; these substances in turn activate additional platelets and promote a prothrombogenic reaction. Activated platelets also release growth factors, such as PDGF, epidermal growth factor (EGF), and transforming growth factor-β, which promote SMC migration and proliferation as well as ECM degradation and ECM protein synthesis. In addition, platelets release monocyte chemoattractants such as platelet factor 4 and β-thromboglobulin, which mediate the recruitment of macrophages to the graft. Platelet deposition and activation continues chronically after graft implantation as evidenced by increased thromboxane levels and decreased systemic platelet counts 1 year after Dacron graft implantation in a canine model,48 and human studies have confirmed platelet adhesion to grafts up to 1 year after implantation.
The deposition and activation of platelets elicit various pathologic cascades; therefore the thrombogenic nature of the synthetic graft surface can lead to both early and late graft failure. A myriad of approaches have been studied to attenuate platelet deposition, aggregation, and degranulation. Antiplatelet agents directly targeting platelet or graft-binding molecules such as platelet surface GPIIb/IIIa and different functional domains of thrombin have been shown to at least transiently decrease the accumulation of platelets on Dacron grafts.49 The surface thrombogenicity of grafts can be altered experimentally through disruption of the blood system’s coagulation cascade on thrombogenic surfaces. We have reported improved patency, thromboresistance, and the absence of intraluminal graft thrombus in heparin-analog–coated ePTFE grafts using a canine bilateral aortoiliac artery model.50 Similarly, combined data tables of clinical data showed a decrease in subacute thrombosis with heparin-coated coronary artery stents.51 Genetic approaches to increase thromboresistance have been used by multiple groups through the overexpression of thrombotic inhibitors, but because quiescent ECs themselves are antithrombotic, there may be limited benefit of this approach compared with a functioning endothelium.
A heparin-bonded Dacron graft from Maquet (Wayne, NJ) is currently available on the market. The heparin is bound primarily through Van der Waals bonds to the polyester fiber pretreated with a cationic agent, tridodecil-methyl-ammonium chloride. The external third of the graft wall is coated with collagen to prevent blood extravasation.52 In a comparative clinical trial involving 209 patients undergoing femoropopliteal bypass grafts, the heparin-bonded Dacron graft exhibited a slightly better but significant (p = 0.04) patency with 1, 2, and 3 years of 70%, 63%, and 55%, compared to untreated ePTFE graft of 56%, 46%, and 42%, respectively.53
A heparin-bonded ePTFE graft developed by W.L. Gore is currently available worldwide. Heparin binding is through covalent end-point attachment of the heparin to the pretreated bioactive surface of the graft. An animal study showed improved patency of the graft compared with the standard ePTFE graft using a canine carotid interposition model up to 6 months.54 The surface heparin activity measured by antithrombin III uptake per unit area was 24.7 ± 7.9 pmol/cm2 at 2 weeks and remained at 15.3 ± 3.7 pmol/cm2 by 12 weeks after implantation. Clinical data to date suggests decent early results55 and 5-year results.56
Whether the anticoagulation works through continuous release of heparin from the material establishing an effective concentration at the interface between blood and the graft surface or through nonconsumptive mechanisms of active function of the heparin immobilized on the material surface is unclear. A major concern with heparinization of the graft surface is the duration of heparin function. Premature release or disturbance of functional heparin or the presence of a physical barrier owing to adherent blood components implies a theoretical inefficacy of the approach. Although theoretically probable,57 there have been discordant case reports on whether these grafts cause heparin-induced thrombocytopenia.58,59
Dacron
PET was first introduced in 1939. DuPont (Wilmington, DE) made further developments and patented its well-known Dacron fiber in 1950.60 Vascular grafts made from Dacron were first implanted by Julian in 195661 and DeBakey in 1958.62
Knitted grafts use a textile technique in which the Dacron threads are looped to form a continuous interlocking chain. Most knitted grafts are manufactured with the threads predominantly oriented in a longitudinal direction (warp knitting) as opposed to a circumferential direction (weft knitting), which has been proved to be structurally unstable. Warp knits have good handling characteristics and reasonable dimensional stability. The loop structure creates greater porosity and radial distension. The velour technique that extends the loops of yarn on the surfaces of the fabrics has been used in the attempt to increase tissue incorporation. An external velour surface permits more extensive and firmer incorporation of the graft into surrounding tissue, but the function of an internal velour structure remains controversial, with the suggestion that it may enhance firm anchorage of the fibrin–platelet pseudointima.63,64
The high porosity of the knitted graft necessitates preclotting to prevent transmural blood extravasation. Gelatin (Vascutek, U.K.) and collagen (Maquet, Wayne, N.J.) are predominantly used to seal knitted Dacron graft pores. The gelatin and collagen in the Vascutek and Maquet grafts are cross-linked by low concentrations of formaldehyde, a method resulting in a weak linkage that allows the gelatin or collagen to be degraded in the body in less than 2 weeks.65,66
Dacron grafts, especially knitted grafts, have been demonstrated to be prone to dilate when implanted into the arterial environment. A 10% to 20% increase in graft diameter size after 1 year following restoration of blood flow is in the expected range. A follow-up study in 95 patients from 2 weeks to 138 months postoperatively (mean, 33 months) demonstrated a mean increase of 17.6% (0% to 84%) compared with initial graft size.67 Woven grafts are structurally more stable. A direct relationship between uncomplicated graft dilatation and structural failure has not been established. Consequently, there is no recommendation on a specific degree of dilation that constitutes a significant hazard and warrants graft replacement.
Structural failure has been reported sporadically in the literature despite advances in the design and manufacturing of grafts (Figure 16-2).
In 1997, an inquiry to the U.S. Food and Drug Administration by Wilson et al.68 revealed 68 cases of Dacron graft failure owing to structure defect, with an average time to failure of 7.4 years (range, 4 to 18 years). The true incidence of structure failure is difficult to estimate because most patients with grafts are not followed-up for long periods or are not being evaluated periodically for graft integrity. A number of factors may contribute to the graft degradation including the design of the textile structure, manufacturing process, surgical handling or application of clamps during implantation, mechanical fatiguing due to cyclic stresses of pulsatile blood flow, and chemical or physical alterations associated with biodegradation. The first generation of double-velour knitted Dacron grafts introduced in the mid 1970s, such as the Microvel and Cooley Double Velour grafts (Boston Scientific Meadox, Oakland, N.J.), showed excessive dilation and rupture after implantation.68,69 It is believed that structural failure of these grafts was related to the fabrication techniques such as the use of trilobal filaments, insufficient strength of guideline yarns, and the weakness of connecting lines where two knitted bands join together to form a tube.67,70 The trilobal filaments were replaced with more durable cylindrical filaments more than 30 years ago.
After implantation, a coagulum containing fibrin, platelets, and blood cells builds up during the first few hours to days and stabilizes over a period of 6 to 18 months, forming a compacted layer. The histologic characteristics observed within Dacron grafts is a compact fibrin layer on the blood contacting surface and densely packed foreign body giant cells between the outer layer of graft wall and surrounding connective tissue capsule. The fibrin layer within the midgraft region beyond 1 to 2 cm from either anastomosis remains acellular regardless of whether the grafts are woven or knitted. Protein impregnation changes the surface properties of Dacron grafts and may induce more inflammatory reaction, but does not change the clinical patency rates of these grafts.65,71,72
ePTFE
The initial host response to ePTFE grafts is similar to that of Dacron grafts.63,64 A fibrin coagulum or amorphous platelet-rich material develops over a time sequence similar in both materials. Lack of luminal surface cellular coverage can be found at the midgraft region for years following human implants.73–75 In outer wrap–reinforced grafts, the wrap delays the infiltration of the cells from perigraft tissue.64
In addition to the standard ePTFE graft, a number of modified grafts have been developed to offer specific properties for various applications. Thin-wall grafts, which have a wall thickness of 0.2 to 0.3 mm versus 0.4 to 0.6 mm for the standard grafts, possess better handling characteristics and improved compliance. The grafts failed to show a better patency for hemodialysis arteriovenous access compared with the standard graft76 and are prone to more postpuncture blood loss. Extended stretch ePTFE graft has longitudinal extensibility that is achieved by a microcrimping of the fibrils. When the stretch graft is extended, the fibrils are extended to their full length, and the microstructure of the graft is then identical to that of the standard ePTFE graft. Stretch ePTFE grafts have somewhat better patency rates when used for atrioventricular access. Unfortunately, no data support such an advantage when used for peripheral reconstructions. Rings or coils are often applied to the abluminal surface of the grafts to provide external support in order to increase the graft resistance to external compression and to reduce kinking.
Still, the rate and extent of tissue ingrowth can be improved by optimizing graft permeability. Clowes et al.77 have demonstrated enhanced tissue ingrowth and complete reendothelialization of 60 µm or 90 µm internodal distance ePTFE grafts in a baboon model.77 Transinterstitial capillary ingrowth was not seen with the more commonly used 30-µm internodal distance ePTFE. Human trials using ePTFE with these expanded internodal distances failed to show any advantage in platelet deposition (considered an indicator of endothelial coverage) compared with standard 30-µm internodal distance ePTFE grafts.78
The delivery of potent angiogens or genes that promote EC-specific mitogenesis or chemotaxis upon injured vessels or prosthetic surfaces may be used to regenerate a rapid and complete endothelium after vascular intervention. We have demonstrated the feasibility of this approach in endarterectomized canine carotid arteries (Figure 16-3).
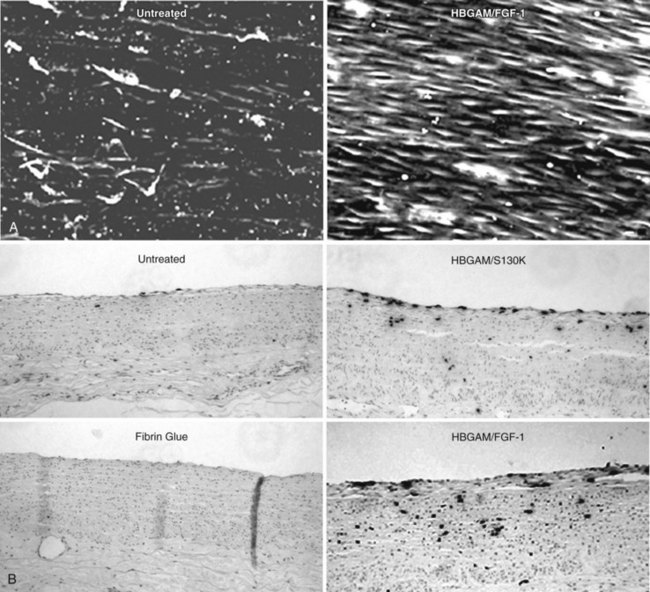
FIGURE 16-3 Endothelial healing with localized delivery of FGF-1 in fibrin glue.
(From Brewster L, Brey EM, Addis M, et al: Improving endothelial healing with novel chimeric mitogens. Am J Surg 192:589–593, 2006.)
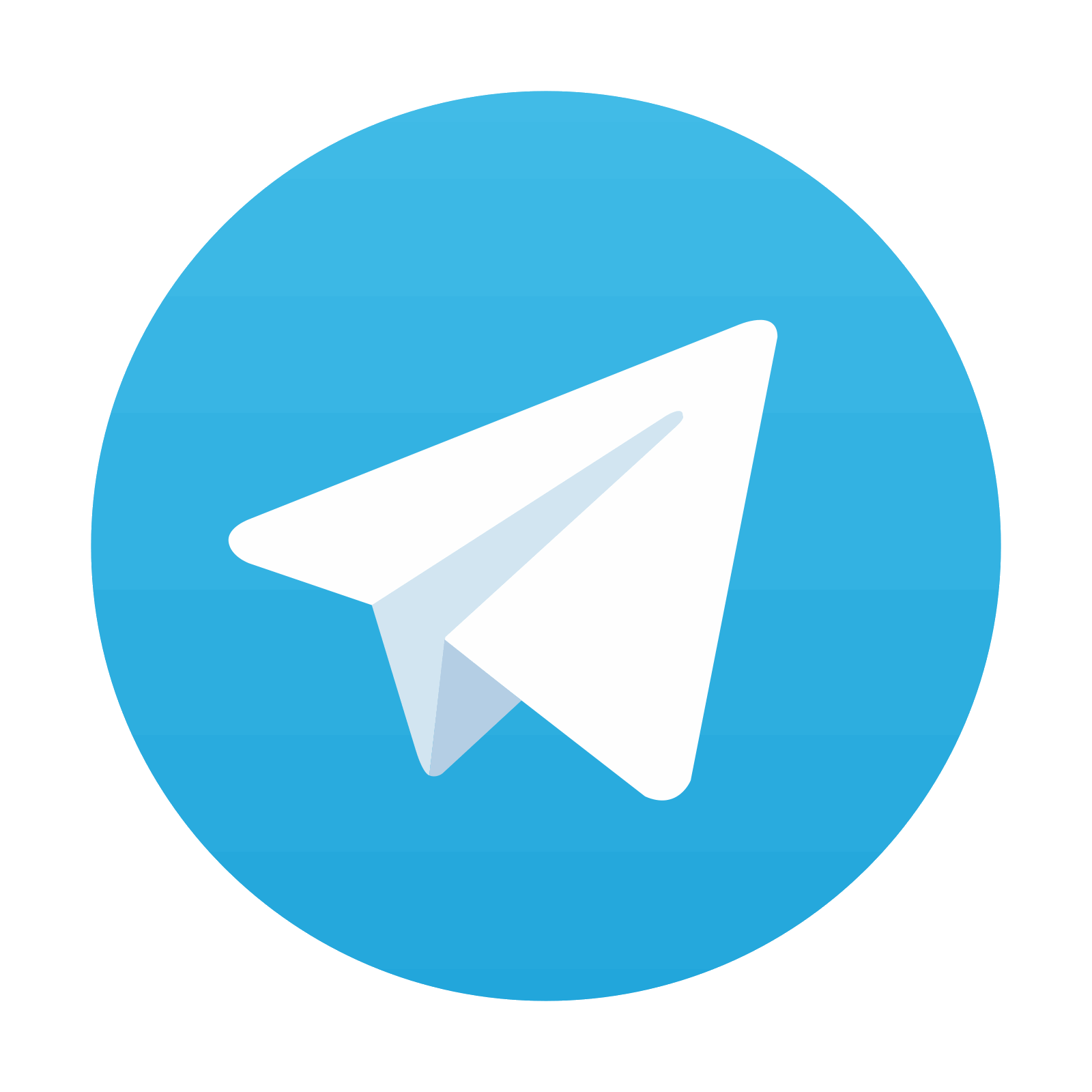
Stay updated, free articles. Join our Telegram channel
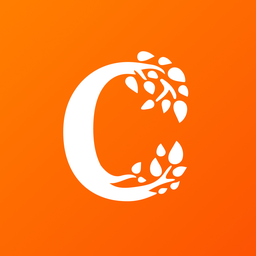
Full access? Get Clinical Tree
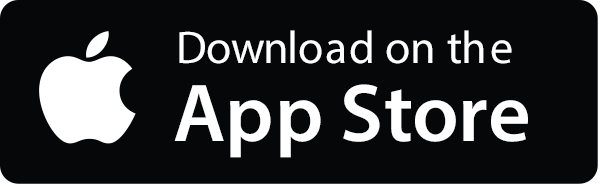
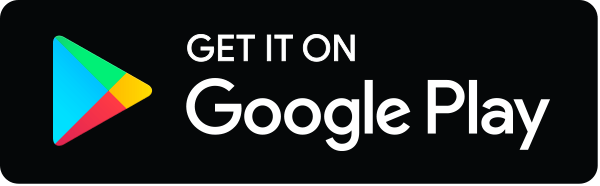