Chapter 1 Vascular Embryology and Angiogenesis
In simple terms, the cardiovascular system consists of a sophisticated pump (i.e., the heart) and a remarkable array of tubes (i.e., blood and lymphatic vessels). Arteries and arterioles (efferent blood vessels in relation to the heart) deliver oxygen, nutrients, paracrine hormones, blood and immune cells, and many other products to capillaries (small-caliber, thin-walled vascular tubes). These substances are then transported through the capillary wall into extravascular tissues where they participate in critical physiological processes. In turn, waste products are transported from the extravascular space back into blood capillaries and returned by venules and veins (afferent vessels) to the heart. Alternatively, about 10% of the fluid returned to the heart courses via the lymphatic system to the large veins.1 To develop normally, the embryo requires delivery of nutrients and removal of waste products beginning early in development; indeed, the cardiovascular system is the first organ to function during morphogenesis.
This chapter summarizes many key molecular and cellular processes and underlying signals in the morphogenesis of the different layers of the blood vessel wall and of the circulatory system in general. Specifically, for intimal development, it concentrates on early EC patterning, specification and differentiation, lumen formation, co-patterning of vessels and nonvascular tissues, and briefly discusses lymphatic vessel development. In the second section, development of the tunica media is divided into subsections examining components of the media, VSMC origins, smooth muscle cell (SMC) differentiation, and patterning of the developing VSMC layers and ECM. Finally, the chapter concludes with a succinct summary of the limited studies of morphogenesis of the blood vessel adventitia. Understanding these fundamental vascular developmental processes are important from a pathophysiological and therapeutic standpoint because many diseases almost certainly involve recapitulation of developmental programs. For instance, in many vascular disorders, mature VSMCs dedifferentiate and exhibit increased rates of proliferation, migration, and ECM synthesis through a process termed phenotypic modulation.2
Tunica Intima: Endothelium
Early Development
Development begins with fertilization of the ovum by the sperm. Chromosomes of the ovum and sperm fuse, and then a mitotic period ensues. The early 16- to 32-cell embryo, or morula, consists of a sphere of cells with an inner core termed the inner cell mass. The first segregation of the inner cell mass generates the hypoblast and epiblast. The hypoblast gives rise to the extraembryonic yolk sac and the epiblast to the amnion and the three germ layers of the embryo known as the endoderm, mesoderm, and ectoderm. The epiblast is divided into these layers in the process of gastrulation, when many of the embryonic epiblast cells invaginate through the cranial-caudal primitive streak and become the mesoderm and endoderm, while the cells that remain in the embryonic epiblast become the ectoderm. Most of the cardiovascular system derives from the mesoderm, including the initial ECs, which are first observed during gastrulation. A notable exception to mesodermal origin is SMCs of the aortic arch and cranial vessels, which instead derive from the neural crest cells of the ectoderm.3
Although ECs are thought to derive exclusively from mesodermal origins, the other germ layers may play an important role in regulating differentiation of the mesodermal cells to an EC fate. In a classic study of quail-chick intracoelomic grafts, host ECs invaded limb bud grafts, whereas in internal organ grafts, EC precursors derived from the graft itself.4 The authors hypothesized that the endoderm (i.e., from internal organ grafts) stimulates emergence of ECs from associated mesoderm, whereas the ectoderm (i.e., from limb bud grafts) may have an inhibitory influence.4 Yet the endoderm does not appear to be absolutely required for initial formation of EC precursors.5,6
The initial primitive vascular system is formed prior to the first cardiac contraction. This early vasculature develops through vasculogenesis, a two-step process in which mesodermal cells differentiate into angioblasts in situ, and these angioblasts subsequently coalesce into blood vessels.7 Early in this process, many EC progenitors apparently pass through a bipotential hemangioblast stage in which they can give rise to endothelial or hematopoietic cells. Furthermore, early EC precursors may in fact be multipotent; there is controversy whether ECs and mural cells share a common lineage.8,9
Following formation of the initial vascular plexus, more capillaries are generated through sprouting and nonsprouting angiogenesis, and the vascular system is refined through pruning and regression (reviewed in10). In the most well studied form of angiogenesis, existing blood vessels sprout new vessels, usually into areas of low perfusion, through a process involving proteolytic degradation of surrounding ECM, EC proliferation and migration, lumen formation, and EC maturation. Nonsprouting angiogenesis is often initiated by EC proliferation, which results in lumen widening.10 The lumen then splits through transcapillary ECM pillars or fusion and splitting of capillaries to generate more vessels.10 In addition, the developing vascular tree is fine-tuned by the pruning of small vessels. Although not involved in construction of the initial vascular plan, flow is an important factor in shaping vascular system maturation, determining which vessels mature and which regress. For instance, unperfused vessels will regress.
Arterial and Venous Endothelial Cell Differentiation
Classically it was thought that arterial and venous blood vessel identity was established as a result of oxygenation and hemodynamic factors such as blood pressure, shear stress, and the direction of flow. However, over the last decade, it has become increasingly evident that arterial-specific and venous-specific markers are segregated to the proper vessels quite early in the program of vascular morphogenesis. For instance, ephrinB2, a transmembrane ligand, and one of its receptors, the EphB4 tyrosine kinase, are expressed in the mouse embryo in an arterial-specific and relatively venous-specific manner, respectively, prior to the onset of angiogenesis.11–13 EphrinB2 and EphB4 are each required for normal angiogenesis of both arteries and veins.12,13 However, in mice homozygous for a tau-lacZ knock-in into the ephrinB2 or EphB4 locus (which renders the mouse null for the gene of interest), lacZ staining is restricted to arteries or veins, respectively.12,13 This result indicates that neither of these signaling partners is required for arterial and venous specification of ECs.
Furthermore, even before initial ephrinB2 and EphB4 expression and prior to the first heart beat, Notch pathway members delta C and gridlock mark presumptive ECs in the zebrafish.14–16 In this model, deltaC is a homolog of the Notch ligand gene Delta, and gridlock (grl) encodes a basic helix-loop-helix protein that is a member of the Hairy-related transcription factor family and is downstream of Notch. The lateral plate mesoderm (LPM) contains artery and vein precursors,17 and prior to vessel formation, the grl gene is expressed as two bilateral stripes in the LPM.16 Subsequently, gridlock expression is limited to the trunk artery (dorsal aorta) and excluded from the trunk vein (cardinal vein).16
In a lineage tracking experiment of the zebrafish LPM, Zhong et al. loaded one- to two-cell embryos with 4,5-dimethoxy-2-nitrobenzyl-caged fluorescent dextran.15 Between the 7- and 12-somite stage of development, a laser was used to activate a patch of 5 to 10 LPM cells with pulsations and thereby “uncage” the dye.15 The contribution of the uncaged cells and their progeny to the dorsal aorta and cardinal vein was assayed the next day.15 Among all the uncaging experiments, marked cells were found in the artery in 20% of experiments and in the vein in 32% of experiments.15 Interestingly, within a single uncaging experiment, the group of marked cells never included both arterial and venous cells, suggesting to the authors that by the 7- to 12-somite stage, an individual angioblast is destined to contribute in a mutually exclusive fashion to the arterial or venous system.15
In addition to being an early marker of arterial ECs, the Notch pathway is a key component of a signaling cascade that regulates arterial EC fate. In zebrafish, down-regulating the Notch pathway through genetic means or injection of messenger ribonucleic acid (mRNA) encoding a dominant-negative Suppressor of Hairless, a known intermediary in the Notch pathway, results in reduced ephrinB2 expression with loss of regions of the dorsal aorta.15,18 Reciprocally, contiguous regions of the cardinal vein expand and EphB4 expression increases.15 By contrast, activation of the Notch pathway results in reduced expression of flt4, a marker of venous cell identity, without an effect on arterial marker expression or dorsal aorta size.15,18 Furthermore, Lawson et al. followed up on these findings to describe a signaling cascade in which vascular endothelial growth factor (VEGF) functions upstream of Notch, and Sonic hedgehog (Shh) is upstream of VEGF.19 Taken together, these results suggest that the Shh-VEGF-Notch axis is necessary for arterial EC differentiation; however, Notch is not sufficient to induce arterial EC fate.
These studies of EC fate raise the issues of when the arterial-venous identities of ECs are specified and whether and/or when these identities become fixed. To examine these issues, Moyon et al. dissected the dorsal aorta, carotid artery, cardinal vein, or jugular vein from the embryonic day 2 to 15 (E2-15) quail and grafted the vessel into the E2 chick coelom.20 On E4, the host embryos were immunostained with arterial-specific antibodies and the quail-specific anti-EC antibody QH1 to determine whether the grafted vessels yielded ECs that colonized host arteries, veins, or neither.20 Quail vessels that were harvested until around E7 and then grafted into the chick colonized ECs in both host arteries and veins, but if harvesting was delayed after E7, plasticity of the grafted vessels decreased.20 Indeed, quail arteries or veins that were isolated after E10 and subsequently grafted almost exclusively contributed to host arteries (> 95% of QH1+ ECs) or veins (~ 90% of QH1+ ECs), respectively.20 Interestingly, when ECs were isolated by collagenase treatment from the quail E11 dorsal aorta wall and then grafted, plasticity of the ECs was restored to that of an E5 aorta (~ 60% of QH1+ EC contribution to arteries and ~ 40% contribution to veins).20 The authors reasoned that an unknown signal from the vessel wall regulates EC identity.20 A recent investigation of the origins of the coronary vascular endothelium also highlights the plasticity of ECs during early mouse development.21 This study suggests that EC sprouts from the sinus venous, the structure that returns blood to the embryonic heart, dedifferentiate as they migrate over and through the myocardium.21 Endothelial cells that invade the myocardium differentiate into the coronary arterial and capillary ECs, while those that remain on surface of the heart will redifferentiate into the coronary veins.21
Endothelial Tip and Stalk Cell Specification in Sprouting Angiogenesis
Tubular structures are essential for diverse physiological processes, and proper construction of these tubes is critical. Tube morphogenesis requires coordinated migration and growth of cells that compose the tubes; the intricate modulation of the biology of these cells invariably uses sensors that detect external stimuli.22 This information is then integrated and translated into a biological response. Important examples of such biological sensors include the growth cones of neurons and the terminal cells of the Drosophila tracheal system. Both of these sensors have long dynamic filopodia that sense and respond to external guidance cues and are critical in determining the ultimate pattern of their respective tubular structures.
Similarly, endothelial tip cells are located at the ends of angiogenic sprouts and are polarized with long filopodia that play both a sensory and motor role22 (Fig. 1-1). In a classic study published over 30 years ago, Ausprunk and Folkman reported that on the day after V2 carcinoma implantation into the rabbit cornea, ECs of the host limbal vessels displayed surface projections that resembled “regenerating ECs,”23 consistent with what is now classified as tip cell filopodia. Tip cells are post-mitotic and express high levels of actin, platelet derived growth factor-β (PDGF-β), and vascular endothelial growth factor receptor-2 (VEGFR-2).22 Proximal to the tip cells are stalk cells that also express VEGFR-2 but, unlike tip cells, are proliferative22 (see Fig. 1-1). During initiation of sprouting angiogenesis, endothelial tip cells develop initial projections prior to stalk cell proliferation.23
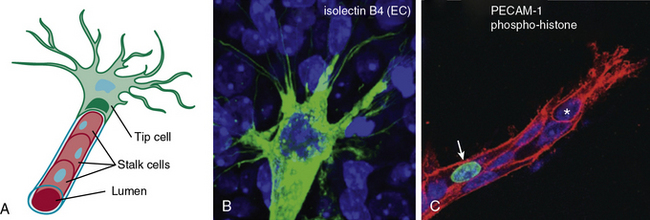
Figure 1-1 Endothelial tip and stalk cells.
(Redrawn with permission from Gerhardt H, Golding M, Fruttiger M, et al: VEGF guides angiogenic sprouting utilizing endothelial tip cell filopodia. J Cell Biol 161:1163–1177, 2003.)
The mouse retina model has been widely utilized in studies of angiogenesis and is an excellent model for studying different aspects of blood vessel development: retinal vasculature is visible externally and develops postnatally through a stereotyped sequence of well-described steps. In addition, at most time points, the retina simultaneously includes sprouting at the vascular front and remodeling at the core. The VEGF pathway is critical for guiding angiogenic sprouts, and in the retina, expression of the ligand VEGF-A is limited to astrocytes, with the highest levels at the leading edge of the front of the extending EC plexus,22 suggesting that the astrocytes lay down a road map for the ECs to follow.24 Vascular endothelial growth factor-A signals through VEGFR-2 on tip and stalk cells. Interestingly, proper distribution of VEGF-A is required for tip cell filopodia extension and tip cell migration, while the absolute concentration, but not the gradient, of VEGF-A appears to be critical for stalk cell proliferation.22
Similar to sprouting angiogenesis, budding Drosophila trachea airways encompass tip cells that lead branch outgrowth and lagging cells that form the branch tube. Ghabrial and Krasnow used this system to address a fundamental question that commonly arises in a variety of disciplines ranging from politics to sports, and in this case to biology: “What does it take to become a leader?”25 An elegant genetic mosaic analysis showed that tracheal epithelial cells are assigned to the role of tip (i.e., leader) or stalk (i.e., follower) cell in the dorsal branch as a result of a competition for FGF activity.25 Those cells with the highest FGF activity become tip cells, and those with lower activity are relegated to the stalk position.25 Furthermore, Notch pathway–mediated lateral inhibition plays an important role in limiting the number of leading cells.25
Similarly, the Notch pathway is also critical in assigning ECs in sprouting angiogenesis to tip and stalk positions (Fig. 1-2; reviewed in26). The Notch ligand Dll4 is specifically expressed in arterial and capillary ECs, and in the developing mouse retina, Dll4 is enriched in tip cells, while Notch activity is greatest in stalk cells.26–28 Attenuation of Notch activity through genetic (i.e., dll4[+/−]) or pharmacological (i.e., γ-secretase inhibitors) approaches results in increased capillary sprouting and branching, filopodia formation, and tip cell marker expression.26,29 Importantly, VEGF appears to induce dll4 expression in vivo; injection of soluble VEGFR1, which functions as a VEGF sink, into the eyes of mice reduces Dll4 transcript levels.29
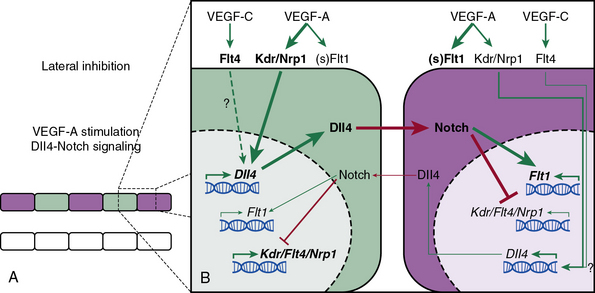
Figure 1-2 Notch-mediated lateral inhibition of neighboring endothelial cells (ECs).
(Redrawn with permission from Phng LK, Gerhardt H: Angiogenesis: a team effort coordinated by notch. Dev Cell 16:196–208, 2009.)
Furthermore, as with the investigations of tip and stalk cells in the Drosophila dorsal airway branches,25 mosaic analyses indicate that competition between cells (in this case for Notch activity) is critical in determining the division of labor in sprouting angiogenesis. Genetic mosaic analysis involves mixing at least two populations of genetically distinct cells in the early embryo, and subsequently comparing the contribution of each cell population to a specific structure or process. Notably, mosaic analysis is usually complementary to experiments with total knockouts and in fact can often be more informative because complete removal of a gene may impair interpretation by grossly distorting the tissue architecture or eliminating competition between cells that harbor differing levels of a gene product.
Experiments using mosaic analysis of Notch pathway mutants in a wildtype background indicate that the Notch pathway acts in a cell autonomous fashion to limit the number of tip cells. In comparison to wildtype ECs in the mouse retina, ECs that are genetically engineered to have reduced or no notch1 receptor expression are enriched in the tip cell population.27
Mosaic studies of Notch signaling components in the developing zebrafish intersegmental vessels (ISVs) are also informative. ISVs traverse between the somites from the dorsal aorta to the dorsal longitudinal anastomotic vessel (DLAV) and are widely used in investigation of blood vessel development. The ISV has been classified as consisting of three (or four) cells in distinct positions: a base cell that contributes to the dorsal aortic cell, a connector cell that courses through the somites, and the most dorsal cell that contributes to the DLAV.30,31 Lateral plate mesoderm angioblasts contribute to the ECs of all the trunk vasculature, including the dorsal aorta, posterior cardinal vein, ISVs, DLAV, and the subintestinal venous vessels. Precursors destined for the ISVs and DLAV initially migrate to the midline dorsal aorta and then between somites to their ultimate positions.30,31 Siekmann and Lawson generated mosaic zebrafish by transplanting into early wildtype embryos marked cells from embryos either lacking the key Notch signaling component recombining protein suppressor of hairless (Rbpsuh) or expressing an activated form of Notch.31 Interestingly, rbpsuh-deficient cells were excluded from the dorsal aorta and enriched in the DLAV position.31 In turn, transplanted cells harboring activated Notch mutations were excluded from the DLAV in mosaics and instead preferentially localized to the base cell and dorsal aorta positions.31
Taken together, the findings indicate that in sprouting angiogenesis, ECs compete for the tip position through Notch-mediated lateral inhibition of neighboring cells26 (see Fig. 1-2). Tip cells express high levels of Dll4, which engages Notch receptors on neighboring cells and thereby inhibits these neighboring cells from developing tip cell characteristics. Furthermore, in the developing retina, the expression of Dll4 is regulated by VEGF-A, which is secreted by astrocytes in response to hypoxia.
Molecular Determinants of Branching
The pattern of many branched structures, such as the vasculature, is critical for function; diverse branched structures use similar signaling pathways to generate their specific patterns. A number of well-studied systems such as the Drosophila trachea, mammalian lung, ureteric bud (UB), and the vasculature consist of hierarchical tubes, progressing from larger to smaller diameter, that transport important gas and/or fluid constituents. The molecular strategies underlying morphogenesis of these patterns often include receptor tyrosine kinase–mediated signaling as well as fine-tuning with inhibitors of these signaling pathways.32,33
In the Drosophila embryo, trachealess selects the trachea primordia and induces conversion of planar epithelium into tracheal sacs that express breathless (btl), the fibroblast growth factor receptor (FGFR) homolog.33,34 The FGF ligand branchless (bnl) is expressed dynamically at positions surrounding the tracheal system, in a pattern which determines where and in which direction a new branch will form.35 Furthermore, loss of bnl prevents branching, and misexpression of bnl induces mislocalized branching.35 Signaling through this FGF receptor pathway is critical for the migration of cells and change in cell shape inherent in formation of primary or secondary airway branches.33,34 Furthermore, tertiary airways consist of a single highly ramified cell whose pattern is not inherently fixed, but instead adapts to tissue oxygen needs in an FGF-dependent manner.36 Finally, as a means of fine-tuning Drosophila airway patterning, branchless induces sprouty, an inhibitor of FGFR signaling, which blocks branching.37,38
Evolutionary conservation of these signaling pathways is striking because the FGF pathway is also essential for determining branch patterning in the mammalian airway system (e.g., the lung). In the mouse, trachea and lung bronchi bud from gut wall epithelium at about E9.5,39 Subsequently, three distinct branching subroutines are repeated in various combinations to generate a highly stereotyped, complex, tree-like structure40 that facilitates gas exchange. In early embryogenesis, the visceral mesenchyme adjacent to the heart expresses FGF10, and FGF10 binds endodermal FGFR2b, the mouse ortholog of Drosophila breathless.32 FGF10 null mice lack lungs and have a blind trachea.41 Similarly, FGFR2b(−/−) mice form underdeveloped lungs that undergo apoptosis.42 Akin to the Drosophila tracheal system, sprouty is a key component of an FGF-induced negative-feedback loop in the lung.38 In response to FGF10, FGFR2b induces Sprouty2 tyrosine phosphorylation and activation, and active Sprouty2 inhibits signaling downstream of FGFR2b.32 In addition, carefully regulated levels of the morphogens sonic hedgehog and bone morphogenic protein (BMP) 4 modulate the branching of lung airways.32
As with the Drosophila and mammalian airway systems, generation of the metanephric kidney requires signals conveyed through epithelial receptor tyrosine kinase. The metanephric mesenchyme secretes glial-derived neurotrophic factor (GDNF), which activates the receptor tyrosine kinase Ret and its membrane-anchored co-receptor Gdnf family receptor alpha 1 (Gfra1), thereby inducing the UB to evaginate from the nephric duct.43,44 These components are required for UB branching because UB outgrowth fails in mice null for Gdnf, Gfra1, or Ret.43 Furthermore, RET is frequently mutated in humans with renal agenesis.45 In addition, FGFR2b is also highly expressed on UB epithelium, and FGFR2b-mediated signaling regulates UB branching.32 FGF7 and FGF10 are expressed in mesenchymal tissue surrounding the UB, and FGFR2b binds with comparable affinity to these ligands.32 As with lung development, BMP4-mediated signaling modulates the branching of the renal system.32
The most well-studied molecular determinants of vascular branching are the VEGF family of ligands (VEGF-A, -B, -C, and -D) and endothelial receptor tyrosine kinases (VEGFR1, 2, and 3).46 VEGF has been shown to be a potent EC mitogen and motogen and vascular permeability factor, and the level of VEGF is strictly regulated in development; VEGF heterozygous mice die around E11.5 with impaired angiogenesis and blood island formation.47,48 During embryogenesis, VEGFRs are expressed in proliferating ECs and the ligands in adjacent tissues. For instance, secretion of VEGF by the ventricular neuroectoderm is thought to induce capillary ingrowth from the perineural vascular plexus.49 Mice null for VEGFR2 or VEGFR1 die around E9.0, with VEGFR2(−/−) mice lacking yolk-sac blood islands and vasculogenesis50 and VEGFR1(−/−) mice displaying disorganized vascular channels and blood islands.51 Although VEGFR3 expression eventually restricts to lymphatic ECs, its broad vascular endothelial expression early in development is critical for embryonic morphogenesis. Indeed, VEGF3 null mice undergo vasculogenesis and angiogenesis; however, the lumens of large vessels are defective, resulting in pericardial effusion and cardiovascular failure by E9.5.52 As with hypoxia-induced FGF-dependent tertiary branching in the Drosophila airway,36 low oxygen levels induce vascular EC branching through hypoxia-inducible factor-1 alpha (HIF-1α)-mediated expression of VEGFR2.53 VEGFR1 is thought to largely function as a negative regulator of VEGF signaling by sequestering VEGF-A. The affinity of VEGFR1 for VEGF-A is higher than that of VEGFR2, and VEGFR1 kinase domain mutants are viable.46
Although generally not as well studied as the role of the VEGF pathway in vessel branching, other signaling pathways, such as those mediated by FGF, Notch, and other guidance factors, are also likely to play important roles. For instance, transgenic FGF expression in myocardium augmented coronary artery branching and blood flow, whereas expression of a dominant-negative FGFR1 in retinal pigmented epithelium reduced the density and branching of retinal vessels.32 Furthermore, a murine homolog of sprouty was shown to inhibit small blood vessel branching and sprouting in mouse embryo cultures.54 The role of the Notch pathway was discussed earlier in the section on endothelial tip and stalk cells. The role of guidance cues initially described in the nervous system is discussed later in the section on neurons and vessels. Finally, the maturation of branches to a more stable state that is resistant to pruning is thought to largely be regulated by signaling pathways that modulate EC branch coverage by mural cells. Interestingly, two of the most important such pathways involve receptor tyrosine kinases such as the angiopoietin-Tie and PDGF ligand receptor pathways.
Vascular Lumenization
Endothelial cells at the tips of newly formed branches lack lumens, but as the vasculature matures, formation of a lumen is an essential step in generating tubes that can transport products. Angioblasts initially migrate and coalesce to form a solid cord that is subsequently hollowed out to generate a lumen through a mechanism that has recently become controversial. Around 100 years ago, researchers first suggested that vascular lumenization in the embryo occurs through an intracellular process involving vacuole formation.55 Seventy years later, Folkman and Haudenschild developed the first method for long-term culture of ECs, and bovine or human ECs cultured in the presence of tumor-conditioned medium were shown to form lumenized tubes (56and references therein). In this and similar in vitro approaches, an individual cell forms Cdc42+ pinocytic vacuoles that coalesce, extend longitudinally, and then join the vacuole of neighboring ECs to progressively generate an extended lumen.56–58 Subsequently, a study using two-photon high-resolution time-lapse microscopy suggested that the lumens of zebrafish ISVs are generated through a similar mechanism of endothelial intracellular vacuole coalescence, followed by intercellular vacuole fusion.59
Recently, however, a number of studies have called this intracellular vacuole coalescence model into question, and instead support an alternate model in which the lumen is generated extracellularly (reviewed in60). One such investigation61 suggests that in contrast to what had been thought previously,30,31 ECs are not arranged serially along the longitudinal axis of the zebrafish ISV, but instead overlap with one another substantially; the circumference of an ISV at a given longitudinal position usually traverses multiple cells. If the lumen of a vessel were derived intracellularly in a unicellular tube, the tube would be “seamless” (as in the terminal cells of Drosophila airways62) and only have intercellular junctions at the proximal and distal ends of the cells. However, in the 30 hours post fertilization zebrafish, junctional proteins zona occludens 1 (ZO-1) and VE-cadherin are co-expressed, often in two medial “stripes” along the longitudinal axis of the ISV, suggesting that ECs align and overlap along extended regions of the ISV.61 Thus, the lumen is extracellular—that is, in between adjacent cells, not within the cytoplasm of a single cell.
In addition, recent investigations show that EC polarization is a prerequisite for lumen formation, and both the Par3 complex and VE-cadherin play a critical role in establishing polarity.63 Endothelial-specific knockdown of β1-integrin reduces levels of Par3 and leads to a multilayered endothelium with cuboidal-shaped ECs and frequent occlusion of midsized vascular lumens.63 VE-cadherin is a transmembrane EC-specific cell adhesion molecule that fosters homotypic interactions between neighboring ECs, and in vascular cords, VE-cadherin is distributed broadly in the apical membrane (reviewed in60). VE-cadherin deletion is embryonic lethal in the mouse; development of VE-cadherin(−/−) embryonic vessels arrests at the cord stage and does not proceed to lumenization.60,64,65 Under normal conditions, during polarization, junctions form at the lateral regions of the apical membrane as VE-cadherin translocates to these regions, which also harbor ZO-1.60 VE-cadherin is required for the apical accumulation of de-adhesive molecules, such as the highly glycosylated podocalyxin/gp135, which likely contributes to lumen formation through cell-cell repulsion. In addition to anchoring neighboring ECs, VE-cadherin also is linked through β-catenin, plakoglobin, and α-catenin to the F-actin cytoskeleton.60
Although establishing polarity of the ECs is a critical step, it is insufficient to induce lumen formation. Indeed, in VEGF-A(+/−) mice, ECs of the dorsal aorta polarize, but this vessel does not lumenize.65 VEGF-A activates Rho-associated protein kinases (ROCKs) that induce nonmuscle myosin II light chain phosphorylation, thereby enhancing recruitment of nonmuscle myosin to the apical membrane.65 Actomyosin complexes at the apical surface are thought to play an important role in pulling the apical membranes of neighboring cells apart, thus generating an extracellular lumen.63
Another important component of the process of EC cord lumenization is the dynamic dissolution and formation of inter-EC junctions. Egfl7 is an EC-derived secreted protein that promotes EC motility and is required for tube formation.66 The knockdown of Egfl7 in zebrafish impairs angioblasts from dissolving their junctions, preventing them from separating, which is required for tube formation.66 Interestingly, a recent study suggests that excessive cell-cell junctions in migratory angioblasts may explain the delayed migration of these cells in endodermless zebrafish.5
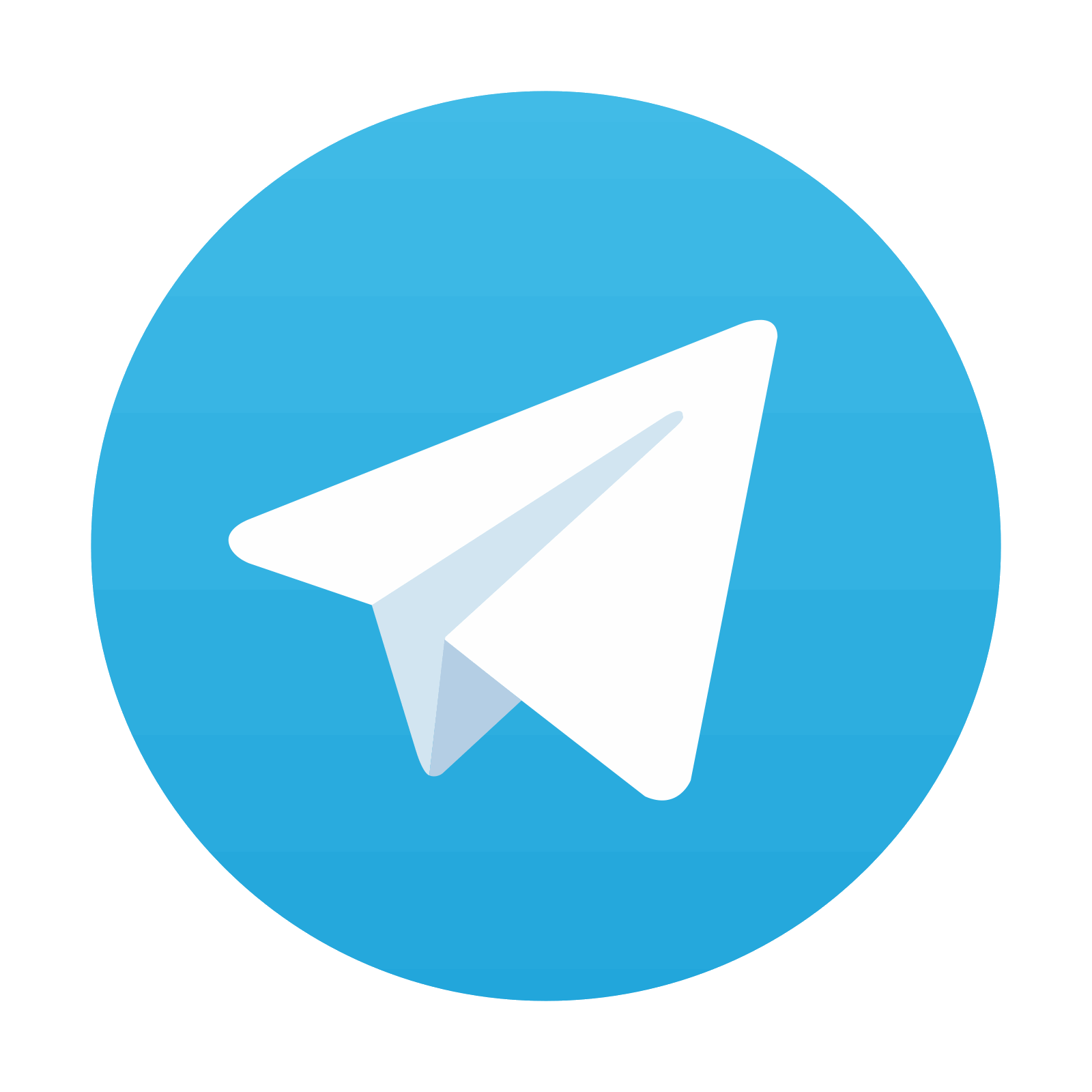
Stay updated, free articles. Join our Telegram channel
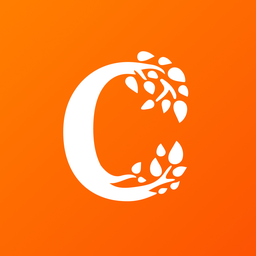
Full access? Get Clinical Tree
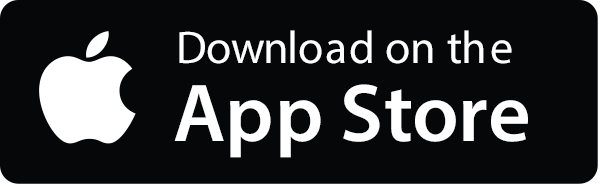
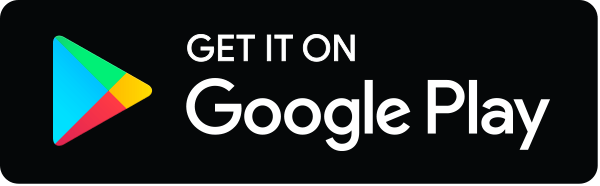