Chapter 14 Computed Tomographic Angiography
Volumetric data acquisition with multidetector computed tomography (MDCT) has enabled the development of computed tomographic angiography (CTA), a diagnostic modality that has clearly revolutionized the diagnosis of vascular disorders. Adequate imaging of the peripheral vascular system during a single acquisition and a single injection of contrast medium became feasible with the introduction of a 4-slice computed tomography (CT) system with a 0.5-second gantry rotation (thinnest collimation 4 × 2.5 mm) in 1998.1 The introduction of 8-slice CT in 2000 enabled shorter scan times, but did not yet provide improved longitudinal resolution (thinnest collimation 8 × 1.25 mm). The introduction of 16-slice CT made it possible to routinely acquire substantial anatomical volumes with isotropic sub-millimeter spatial resolution.2 Computed tomographic angiography scans of the peripheral vasculature could now be routinely acquired with 16 × 0.625 mm or 16 × 0.75 mm collimation, which provided the level of resolution required to investigate small-vessel involvement (<1 mm).
Fundamentals of Computed Tomography Imaging
Major Components of a Computed Tomography Scanner
The major components of a CT scanner are an x-ray tube and generator, a collimator, and photon detectors. These components are mounted on a rotating gantry where the x-ray tube produces the x-rays necessary for imaging. The pre-detector collimator helps shape the x-ray beams that emanate from the x-ray tube in order to cut out unnecessary radiation. The detectors consist of multiple rows of detector elements (>900 elements per row in current scanners) that receive x-ray photons that have traversed through the patient, with the post-detector collimators preventing backscatter, which degrades image quality. Newer scanners have as many as 320 detector rows, and the width of each detector (“detector collimation”) has decreased from 2.5 mm in 4-slice systems to 0.5 mm. The most important benefit of increasing the detector rows is increased coverage per gantry rotation (a 320-row detector CT with a detector width of 0.5 mm will have 160 mm z-axis coverage). The submillimeter detector width improves spatial resolution in the z-axis, while increased coverage shortens scan time. Each detector element contains radiation-sensitive solid state material (e.g., cadmium tungstate, gadolinium oxide, gadolinium oxysulfide) that converts the absorbed x-rays into visible light.3 The light is then detected by a silicone photodiode, amplified, and converted into a digital signal.
Gantry rotation time determines the temporal resolution of the images, with older scanners having a rotation time of 0.75 seconds, and more contemporary scanners a rotation time of 0.33 seconds.2 The temporal resolution of a single source scanner, one x-ray generator mounted on the gantry, is slightly higher than half the time it takes for the gantry to rotate 360 degrees. Thus a 0.33-second gantry rotation will effectively provide a temporal resolution of 0.17 seconds. With a dual-source scanner, two x-ray generators mounted on the gantry, the temporal resolution will improve by a factor of two.
Electrocardiographic Gating
Electrocardiographic gating is a method of gating imaging events to portions in the cardiac cycle where motion may be minimal, namely diastole. Electrocardiographic gating is indispensable for coronary imaging and vascular structures that are prone to cardiac motion artifact, such as the ascending aorta. The two most common ECG gating methods are retrospective and prospective gating. With traditional spiral scanning, ECG gating is performed retrospectively, where the data and ECG information are acquired, and subsequent reconstructions can be performed at various time points in the R-R interval.4 Compared to prospective ECG gating, which is the method used for axial scanning, the scan is triggered at the R wave, and image acquisition occurs at a fixed point in the cardiac cycle. However, with recent advances in CT imaging, it is now possible to perform a prospectively ECG-triggered helical scan using high pitch with extremely low radiation exposure.5 These have been referred to as flash scans and are gaining significant popularity for coronary imaging.
Contrast Administration
All angiographic x-ray contrast remains in the extracellular space and rapidly distributes between the intravascular and extravascular spaces immediately after intravenous (IV) administration.6 It is the process during the early phase of rapid contrast distribution and redistribution that determines vascular enhancement. Vascular enhancement differs significantly from parenchymal (soft tissue) enhancement characteristics. The two key components that determine arterial enhancement are the amount of contrast per unit time (mL/s) and the duration of administration (seconds). The resulting product of the two is the volume of contrast (flow rate × duration). For example, 100 mL of contrast media given at 5 mL/s will require 20 seconds to deliver. The relationship between flow rate, volume of contrast, and duration of administration is the most important concept to understanding injection protocols for vascular imaging.
Currently, low or isoosmolar nonionic contrast agents are the most commonly used for CTA. It is imperative to assess renal function prior to administration of contrast so decisions can be made in regard to prophylactic measures, type of contrast used, and whether the study should be cancelled. Contrast is given IV using a power injector. Since contrast arrival time to the region of interest may vary, appropriate timing must be determined by using a test bolus or automated bolus tracking technique.7 The less common technique of using a test bolus is performed by giving a small dose of contrast material and determining the time it takes for the region of interest to opacify. More commonly, a triggered or automated bolus tracking technique is used, where a region of interest is drawn on the aorta closest to the area of interest. A repetitive low-dose acquisition is acquired 5 to 10 seconds after contrast administration until a given HU threshold is achieved (typically 110 HU). The actual CTA will be acquired once this threshold is obtained. The typical volume of contrast used is 100 to 120 mL, with an iodine concentration between 320 and 370 mg/mL, administered at a rate of 4 mL/s and followed by a saline flush.
Image Reconstruction at the Scanner Console
Various image reconstruction filters are offered by each manufacturer. Filters are referred to as sharp or soft. Sharper reconstruction filters will provide more details but also more noise and are best for assessment of stents and areas of calcification. Softer reconstruction filters provide less image detail but less noise as well. Soft to medium filters are usually used for most CTA applications. Image reconstruction can also be performed at different cardiac phases of cardiac-gated acquisitions. It may be important in assessment of coronary anatomy in cases of thoracic aortic dissection and thoracic aortic aneurysms.8 This is most important for cardiac CTA where coronary anatomy may have to be assessed at different phases to ensure accurate delineation of coronary stenosis. When ECG-gated thoracic aortic imaging is performed, various phases can be reconstructed to assess the aorta.
Image Postprocessing
Similar to vascular magnetic resonance angiography (MRA) (see Chapter 13), multiple postprocessing techniques can be used in vascular CTA to assess the hundreds to thousands of images generated. Usually two data sets are reconstructed: thick and thin sets. The thick set (5.0 mm) is used for general assessment, whereas the thin set (0.5-0.75 mm) is better suited for detailed evaluation. Image formats used for evaluation include (1) multiplanar reformats (MPR), (2) maximal intensity projections (MIP), (3) curved planer reformats (CPR), (4) volume rendering (VR), and (5) shaded surface display (SSD). Please refer to Chapter 13 for a description of these techniques.
Radiation Exposure and Radiation Dose Reduction
Radiation exposure of the patient during CT and the resulting potential radiation hazards has recently gained considerable attention from both the public and the scientific literature.9–12Radiation exposure is defined as the total charge of ions produced in a unit of dry air by a given amount of x-ray or γ-ray irradiation. In the International System of Units (SI), exposure is measured in terms of coulombs (C)/kg or amperes (A) .s/kg. Exposure is also commonly measured in units of roentgens (R), where 1 R equals 2.58 × 10− 4 C/kg. Absorbed dose is the energy imparted to a volume of matter by ionizing radiation, divided by the mass of the matter. The SI unit of absorbed dose is the gray (Gy), where 1 Gy equals 1 joule (J)/kg. The traditional unit is the rad, short for radiation absorbed dose, which equals 1 cGy or 10− 2 Gy. Although absorbed dose is a useful concept, the biological effect of a given absorbed dose varies depending on the type and quality of radiation emitted. A dimensionless radiation weighting factor is used to normalize for this effect, where the weighting factor ranges from 1 for photons (including x-rays and γ-rays) and electrons to 20 for α particles. The sievert (Sv), a special SI unit to represent equivalent dose, was adopted to avoid confusion with absorbed dose. One Sv equals 1 J/kg. The traditional unit for equivalent dose is the rem, short for roentgen equivalent man. One rem equals 1 cSv, or 10− 2 Sv. Equivalent dose multiplied by the tissue-weighting factor is often termed weighted equivalent dose, properly measured in Sv or rem. The sum of the weighted equivalent dose over all organs or tissues in an individual is termed the effective dose (E).13
Computed Tomography–Specific Dosimetry
In addition to the nomenclature for radiation dosimetry described above, a particular set of terms has been developed for CT.14 The dose profile (D[z]) for a CT scanner is a mathematical description of the dose as a function of position on the z axis (perpendicular to the tomographic plane). The CT dose index (CTDI), measured in Gy, is the area under the radiation dose profile for a single rotation and fixed table position along the axial direction of the scanner, divided by the total nominal scan width or beam collimation. CTDI is difficult to measure and therefore not commonly reported; instead, the CTDI100 is measured. CTDI100 represents the integrated radiation exposure from acquiring a single scan over a length of 100 mm. To estimate the average radiation dose to a cross-section of a patient’s body, a weighted CTDI (CTDIw) is calculated. This is determined by the equation:
where EDLP, measured in units of mSv / (mGy ∙ cm), is a body region–specific conversion factor. The most commonly used EDLP values are reported in the 2004 CT Quality Criteria.15 These EDLP values are determined using Monte Carlo methods averaged for multiple scanners.
Dose Reduction Techniques
There are several ways to lower the dose delivered to a patient.14,16 These methods can be used either in isolation or combined to exponentially lower exposure. Reducing tube current will lead to a direct reduction in radiation dose to a patient. However, a conscious decision must be made as to whether the tradeoff in radiation reduction outweighs image quality. This becomes very important in obese patients, where reduction in tube current may result in rather poor images. The contrast-to-noise ratio increases with decreasing x-ray tube voltage.5 As a consequence, to obtain the adequate contrast-to-noise ratio, the dose to the patient may be reduced by lowering the kV setting. There is nearly a 50% reduction in radiation exposure when using 80 kV instead of 120 kV when performing CTA. A recent study recommends 100 kV as the standard mode for aortoiliac CTA, and reports dose savings of 30% without loss of diagnostic information.17
Electrocardiographic-controlled dose modulation is a method employed during continuous imaging with retrospective studies. Typically the output is kept at its nominal value during a user-defined phase (in general, the mid- to end-diastolic phase), while during the rest of the cardiac cycle, the tube output is reduced to 20% of its nominal value to allow for image reconstruction throughout the entire cardiac cycle. Using this technique, dose reduction of 30% to 50% has been demonstrated in clinical studies.18–22
Anatomical tube current modulation is a technique adapted to the patient’s geometry during each rotation of the gantry. Tube output is modulated on the basis of the tissue attenuation characteristics of the localizer scan or determined online by evaluating the signal of a detector row. By employing such a technique, dose can be reduced by 15% to 35% without degrading image quality, depending on the body region.23 A more sophisticated variation of anatomical tube current modulation varies tube output according to patient geometry in the longitudinal direction so as to maintain an adequate dose when moving to different body regions—for instance, from thorax to abdomen (automatic exposure control).23 Automatic adaptation of the tube current to patient size prevents both over- and under-irradiation, considerably simplifies the clinical workflow for the technician, and eliminates the need to look up tables of patient weight and size for adjusting the mAs settings.
Clinical Applications for Computed Tomographic Angiography in Vascular Disease
Computed Tomographic Angiography of the Neurovascular Circulation
Technical considerations
Computed tomographic angiography is comparable to digital subtraction angiography (DSA) for the measurement of residual carotid artery stenosis and is the preferred method of assessment at many institutions.24–26 To perform CTA of the head and neck arteries, the patient is placed in the supine position with the arms along the sides of the body. The topogram is used to assist planning of the imaging volume, which should start from the aortic arch and end at the level of the circle of Willis. A submillimeter detector collimation is required for images, with the greatest spatial resolution in the z axis. A test bolus or bolus tracking algorithm can be used to determine the start of the scan. The pitch can range from 0.5 and 1.0, depending on the vendor and the number of detector rows. Breath-holding and cessation of swallowing is critical to eliminate motion artifacts. For assessment of carotid circulation, reconstruction is typically performed with smooth reconstruction kernels (e.g., Siemens B20f) using a slice thickness between 0.6 and 1.0 mm and a 50% to 80% reconstruction increment.
Attention to appropriate window settings will have significant impact on measured variances in luminal contrast density. Differences in the measured residual lumen and beam-hardening from calcified plaque will overestimate the degree of stenosis. To avoid this problem, a simple formula may be used to calculate the optimal window settings for carotid stenosis assessment with CTA.27 The window width used is the product of the intraluminal HU × 2.07, and the widow level is the product of the intraluminal HU × 0.72. The degree of stenosis should be reported in terms of percent stenosis or residual luminal area. Percent stenosis is defined as the ratio of the maximal luminal narrowing to the normal internal carotid artery (ICA) distal to the bulb, as was described in the North American Symptomatic Carotid Endarterectomy Trial (NASCET).28 However, the segment of normal ICA measurement can range from 5 to 8 mm, which will affect the calculated degree of stenosis. This can be averted by using the residual luminal diameter instead of the percent stenosis,29–33 or using a simple visual estimation of the degree of stenosis rather than using a caliper-based method.34 The current standard uses the percent stenosis measurement based on NASCET, but there are papers describing the use of residual lumen diameter, where 1.5 mm is used as the cutoff for hemodynamically significant stenosis, which correlates with an ultrasound peak systolic velocity of greater than 250 cm/s and a NASCET measurement of more than 70% stenosis.35
If the clinical question is whether there is a total or subtotal internal carotid occlusion, an immediate delayed acquisition through the neck is helpful to detect slow opacification through a residual lumen. It is important to be aware that sometimes the ascending pharyngeal artery may mimic a subtotally occluded ICA. This is easily differentiated by the fact that the ascending pharyngeal artery does not reach the skull base, whereas the ICA does. Accurate distinction is critically important; a subtotally stenosed ICA may be amenable to revascularization.36–38
Computed Tomographic Angiography in Atherosclerotic and Nonatherosclerotic Disease
Technical advances in CTA from the last decade have allowed unprecedented imaging for a number of neurovascular applications, including evaluation for carotid artery stenosis, acute ischemic and hemorrhagic stroke, intracranial vascular anomalies, and craniocervical trauma. By far the most common indication for CTA of the extracranial circulation is for suspected carotid artery stenosis due to atherosclerosis (Fig. 14-1). Computed tomographic angiography is also part of the comprehensive evaluation of the patient with an acute stoke, where nonenhanced brain CT, vascular angiography, and perfusion imaging can be acquired during the comprehensive CT examination. Nonatherosclerotic diseases such as fibromuscular dysplasia (FMD), aneurysms or pseudoaneurysms, or dissections can also be imaged with high spatial resolution (Fig. 14-2). Additionally, CTA has a unique role in follow-up after carotid artery stenting procedures (see Fig. 14-1) instead of using MRA, which typically has significant susceptibility artifacts.
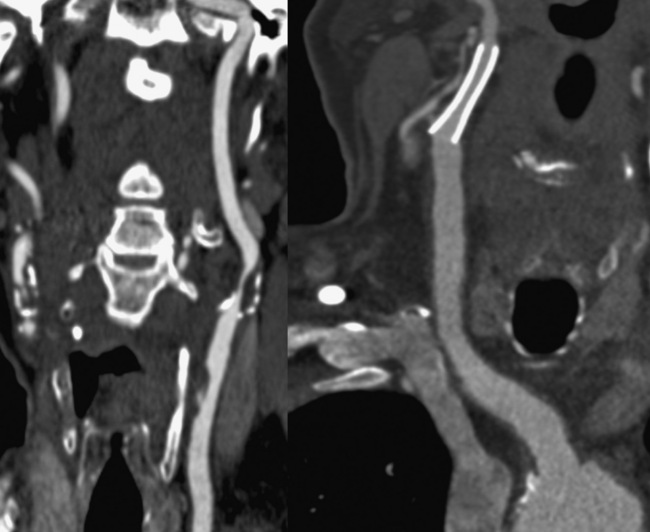
Figure 14-1 Carotid artery atherosclerosis.
(Adapted with permission from Berg M et al: CT angiography of the extracranial and intracranial circulation with imaging protocols. In Mukherjee D, Rajagopalan S, editors: CT and MR angiography of the peripheral circulation: practical approach with clinical protocols, London, 2007, Informa UK Ltd., p. 67.)
Diagnosis of carotid disease
MDCT has shown a 100% correlation with invasive angiography for locating significant stenosis. The interobserver agreement in evaluating total versus subtotal occlusion, stenosis length, retrograde ICA flow, and location of the stenotic site is 1.0, 0.94, 0.86, and 0.89, respectively.24,39 MDCT is also helpful in identifying underlying etiology such as dissection, atherosclerosis, or thrombosis. Berg et al. studied 35 consecutive symptomatic patients with cerebrovascular disorders (e.g., minor stroke, transient ischemic attack (TIA), amaurosis fugax, dizziness) and performed MD-CTA.24 The main focus of the study was comparing MD-CTA to conventional x-ray DSA and rotational DSA as reference standards. In this study, the degree of stenosis was slightly underestimated with CTA, with mean differences (± standard deviation) per observer of 6.9 ± 17.6% and 10.7 ± 16.1% for cross-sectional, and 2.8 ± 19.2% and 9.1 ± 16.8% for oblique sagittal MPRs, compared with x-ray and rotational angiography, respectively. Computed tomographic angiography was somewhat inaccurate for measuring the absolute minimal diameter of subtotally occluded carotid arteries. For symptomatic lesions, interactive CTA interpretation combined with MPR measurements of lesions with a visual estimate of 50% or greater diameter narrowing had a sensitivity of 95% and specificity of 93% for detection of carotid stenosis compared with DSA. Based on these data, it can be concluded that CTA is sensitive for detecting significant carotid artery stenosis.
Computed Tomographic Angiography of the Thorax
Pulmonary arteries
Technical Considerations and Clinical Applications
Pulmonary embolism (PE) is the third most common cause of cardiovascular death in the United States after ischemic heart disease and stroke, with an estimated annual incidence of 300,000 to 600,000 cases per year.40 Even though there is a high prevalence of PE, it continues to be underdiagnosed, with only 43 to 53 patients per 100,000 being accurately identified.41
Computed tomography of the pulmonary arteries (CTPA) is the current diagnostic test of choice for assessing pulmonary thromboembolic disease. In the PIOPED-II study,42 CTPA was principally performed on 4-slice CT with a slice thickness of 1 to 1.25 mm. In this study, the overall positive predictive value in diagnosing PE was 86% (97% for proximal, 68% for segmental, and 25% for subsegmental thrombus), and the negative predictive value was 95%. The value of CTPA varied with the clinical pretest probability of PE: in patients with high or intermediate clinical probability, the positive predictive value for PE was 96%. However, in the face of low clinical pretest likelihood, 42% of patients had a false-positive CTPA result. Therefore, a positive CTPA that was discordant with clinical data had little diagnostic value, at least on the basis of this study. There are randomized controlled studies using later-generation CT scanners that have addressed the issue of their superiority over the 4-slice systems used in PIOPED-II.43–45 The expectation is that thinner detectors and larger detector assembly would allow rapid imaging of the pulmonary artery and branches in a few seconds, avoiding motion artifact.
The CTPA findings of acute PE can be divided into arterial findings and ancillary findings. Intraluminal filling defects may partially or completely occlude a pulmonary artery and typically cause significant dilation of the vessel. Acute emboli appear as adherent intravascular filling defects that form acute angles to the vessel wall, whereas chronic thrombi have the appearance of mural adherent thrombi contiguous with the vessel wall. Acute PEs that straddle the bifurcation of the left and right pulmonary arteries are referred to as saddle emboli (Fig. 14-3). Lung infarcts, atelectasis, and oligemia of the affected territory are common lung parenchymal findings with a PE.
< div class='tao-gold-member'>
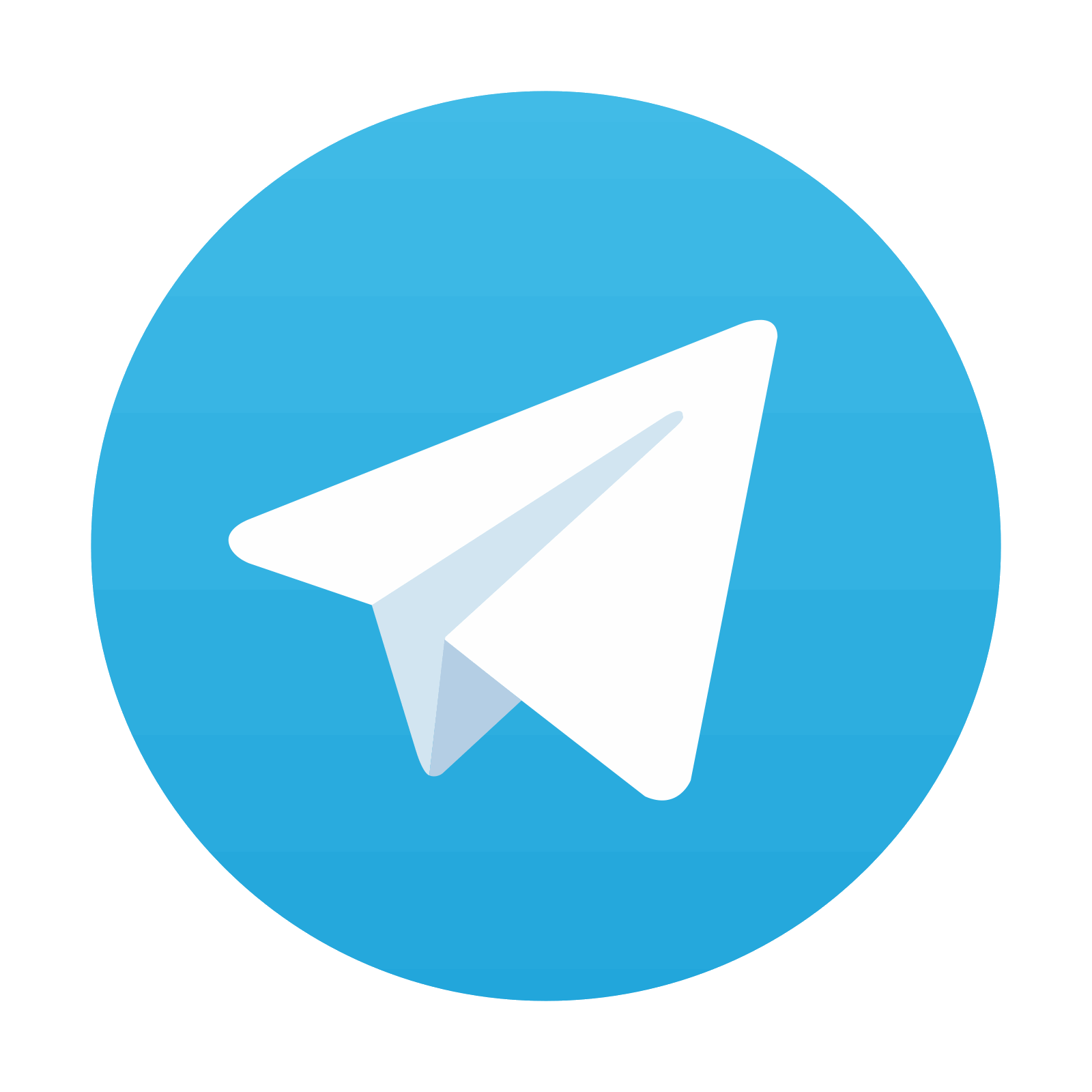
Stay updated, free articles. Join our Telegram channel
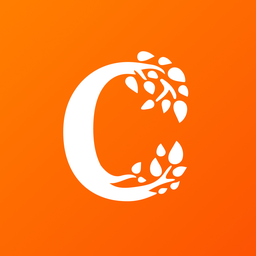
Full access? Get Clinical Tree
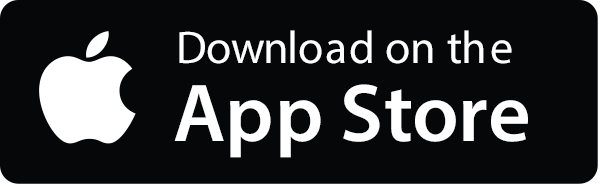
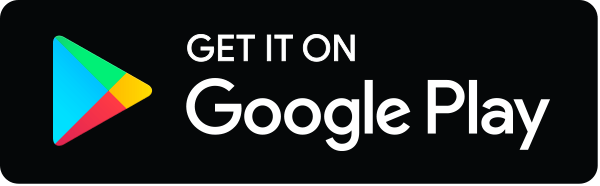