Background
Although multiple echocardiographic methods exist to calculate cardiac output (CO), they have not been validated in mice using a reference method.
Methods
Echocardiographic and flow probe measurements of CO were obtained in mice before and after albumin infusion and inferior vena cava occlusions. Echocardiography was also performed before and after endotoxin injection. Cardiac output was calculated using left ventricular volumes obtained from an M-mode or a two-dimensional view, left ventricular stroke volume calculated using the pulmonary flow, or estimated by the measurement of pulmonary velocity time integral (VTI).
Results
Close correlations were demonstrated between flow probe-measured CO and all echocardiographic measurements of CO. All echocardiographic-derived CO overestimated the flow probe-measured CO. Two-dimensional image-derived CO was associated with the smallest overestimation of CO. Interobserver variability was lowest for pulmonary VTI-derived CO.
Conclusion
In mice, CO calculated from two-dimensional parasternal long-axis images is most accurate when compared with flow probe measurements; however, pulmonary VTI-derived CO is subject to less variability.
Mice are used extensively in cardiovascular research, and evaluation of their cardiac output (CO) provides important information on their cardiac function. CO in mice can be directly measured using a Doppler flow probe positioned on the ascending aorta or calculated from the left ventricular (LV) diastolic and systolic volumes measured by an intraventricular conductance catheter. Although these techniques have been extensively used, they are invasive and time-consuming, and their performance disrupts the normal physiologic conditions. Serial follow-up of the CO using these techniques has not been attempted. Similarly, measurement of the CO using transesophageal echocardiography has been described; however, this technique necessitates deep anesthesia and lengthy analysis. Magnetic resonance imaging also allows quantification of mice ventricular volumes, and therefore potentially of CO. Serial imaging of a large number of animals using magnetic resonance imaging is difficult because of the length of acquisition, processing, and expense.
Transthoracic echocardiography is increasingly used to assess mice cardiac phenotypes. Transthoracic echocardiograms are relatively easy to perform and allow noninvasive serial measures of LV size and function. Multiple approaches have been proposed to calculate CO using echocardiography in humans. CO may be similarly calculated in mice using transthoracic echocardiography. Feldman et al. reported that CO calculated using an M-mode method did not correlate to that measured with a flow probe; of note, however, the approaches were not simultaneous because echocardiography was performed closed-chest. Other routinely used echocardiographic approaches to CO have not been validated and compared using a reference method in mice. Such validation is important because the mouse heart’s size, shape, and beating rate may invalidate techniques used in humans and large animals.
The objective of this study was to determine whether echocardiography is a reliable method to measure mouse CO and what echocardiographic approach is most appropriate for this measurement. For this purpose, CO measured or estimated by a variety of echocardiographic methods (using the M-mode, two-dimensional images, and pulmonary flow) was compared with CO measured by an ascending aortic flow probe. The variability of each method was also tested.
Materials and Methods
Protocol
C57BL/6 male mice (25 ± 2 g) were anesthetized with an intraperitoneal injection of ketamine (0.1 mg/g) and xylazine (0.01 mg/g) and placed supine on a heated operating table. After tracheotomy, mice were ventilated and a custom-made catheter (PE10, Becton Dickinson, Franklin Lakes, NJ) was inserted via the right carotid artery to monitor mean arterial pressure. A venous line was inserted in the left jugular vein. A thoracotomy was performed, and a flow probe (1.5SL; Transonic Systems, Ithaca, NY) was placed around the ascending aorta. The probe was precalibrated in the manufacturer’s factory under controlled procedures traceable to the National Institute of Standards and Technology. This validation is done using a phantom with a roller pump and controlled liquid flows through customized tubing that does not interfere with the flow probe signal. The calibration is done every year by the manufacturer. CO was continuously displayed and recorded with a digital data acquisition system (DI720; Dataq Instruments, Akron, OH). In this well-controlled open-chest model, transthoracic echocardiography was performed in the decubitus position using a transducer centered on 30 MHz (RMV707B, Vevo 770, Visualsonics, Toronto, Ontario, Canada) with an axial resolution of 55 μm and a lateral resolution of 115 μm at a depth of 1.3 cm. Both the flow probe and the echocardiography probe were held using mechanical arms. Because of frequency overlap between both ultrasound systems, Doppler echocardiographic and flow probe data could not be recorded simultaneously. The flow probe measurement was performed first and immediately followed by the echocardiographic measurement. Data were measured during steady-state conditions using an intravenous infusion of albumin (12.5% in normal saline, 50 μL/min) and during CO decrease obtained by intermittent clamping of the inferior vena cava. The ventilator was turned off, and measurements were obtained at end expiration. The flow probe-measured CO was averaged over five consecutive cardiac cycles.
Echocardiographic Measurements of CO
Three echocardiographic algorithms for CO measurement were compared with flow probe-measured CO ( Figure 1 ). In methods 1 and 2, CO was calculated as the product of LV stroke volume (SV = LV end-diastolic volume- LV end-systolic volume) and heart rate. In method 3, CO was calculated as the product of pulmonary flow/cardiac cycle and heart rate. Method 1: LV volumes derived from D 3 (M-mode). LV end-diastolic and end-systolic volumes were calculated from an M-mode image of a parasternal short-axis view at the level of the papillary muscles by the “D 3 ” formula. Left ventricular end-diastolic diameter (LVEDD) and left ventricular end-systolic diameter (LVESD) were measured, and SV was derived from the difference of LV end-diastolic and end-systolic volumes calculated respectively as LVEDD 3 and LVESD 3 ( Figure 1 A). CO was calculated as the product of SV and heart rate. Method 2: LV volumes derived from the area-length formula (parasternal long-axis view). The second method used a parasternal long-axis two-dimensional view. LV end-diastolic and end-systolic volumes were calculated using a prolate-ellipsoid formula (volume = 8.A 2 /3π.L, A = LV area, and L = LV length), as shown in Figure 1 B. SV was then calculated as the difference between these two volumes. Method 3: Pulmonary flow. The third method calculated the pulmonary flow as the product of the main pulmonary area at the level of the pulmonary valve tips and the velocity time integral of the pulsed-wave Doppler of the pulmonary flow (VTI) at that level ( Figure 1 C). Both pulmonary measurements were obtained from a parasternal short-axis view at the aortic root level. The pulmonary flow VTI was also compared with flow probe results. All measurements were obtained by one observer blinded to the results of the flow probe and averaged on five consecutive cardiac cycles. The echocardiographic-derived M-mode measurements were obtained in 9 mice (36 measurements), the two-dimensional measurements were obtained in 6 mice (33 measurements), and the pulmonary measurements were obtained in 9 mice (34 measurements).

Ratio of the Long to Short Axis of the LV in Mice
To determine a possible cause of under- or overestimation of SV and CO measurements by echocardiography, the ratio of the long axis to short axis was measured in the mice undergoing the two-dimensional echocardiographic measures and in five additional closed-chest control mice. These five control mice (C57BL6 2-month-old male) were sedated using isoflurane 0.5% to 1%, and a parasternal long-axis view was obtained.
Application to Endotoxinic Shock
A baseline echocardiogram was performed in 10 C57BL6 2-month-old male mice. Measurements of CO were obtained using M-mode, two-dimensional images, and pulmonary flow as described previously. Three days later, the mice were challenged with an intraperitoneal injection of Escherichia coli 0111:B4 endotoxin (LPS, 25 mg/kg). An echocardiogram was repeated 5 hours after the injection. To avoid hypothermia and dehydration, the mice were kept on a heated platform and received 0.5 mL of saline intraperitoneally 30 minutes before the echocardiogram.
Statistical Analysis
Statistical analysis was performed using JMP 9.0 (SAS Institute Inc., Cary, NC). Results are reported as mean ± SEM. The prediction of flow probe-measured CO in our experiments was first obtained by a standard least-squares model including the effect of the echocardiographic parameter of interest, the effect of the mouse, and the crossed effect of mouse∗echocardiographic parameter. To compare the ability of each echocardiographic algorithm to predict flow probe-measured CO, the SD of the residuals was calculated for each algorithm. The amplitude of the overestimation of the flow probe-measured CO by echocardiographic methods was compared using analysis of variance and Student t test if appropriate. The comparison of the echocardiographic parameters before and after endotoxin was obtained using Student paired t tests. A value of P < .05 was considered significant. The intraobserver and interobserver variability of each echocardiographic method was expressed as mean ± SD of the difference between two measurements. The variability between methods was compared using a test for equality of variances. If the test for equality of variances showed an overall difference between groups, post hoc pairwise comparison was performed.
Results
Hemodynamic Measurements at Baseline
At baseline with all catheters and flow probe in place, systolic arterial pressure was 70 ± 4 mm Hg and heart rate was 366 ± 19 bpm in the mice used for M-mode measurements, 72 ± 6 mm Hg and 445 ± 24 bpm in the mice used for two-dimensional measurements, and 72 ± 7 mm Hg and 373 ± 22 bpm in the mice used for pulmonary measurements.
Relationship between Echocardiographic- and Flow Probe-Measured CO
CO measured by flow probe correlated closely to CO calculated using M-mode (flow probe-measured CO = [0.38 M-mode-calculated CO] + 0.78, r 2 = 0.78, P < . 0001, Figure 2 A), two-dimensional images (flow probe-measured CO = [0.64 two-dimensional-calculated CO] + 0.9, r 2 = 0.87, P < . 0001, Figure 2 B), and pulmonary flow (flow probe-measured CO = [0.48 pulmonary flow-calculated CO] + 0.84, r 2 = 0.78, P < . 0001, Figure 2 C). There was also a close correlation between CO measured by flow probe and estimated by the pulmonary velocity time integral (VTI) (flow probe-measured CO = [2.34 VTI-derived CO] – 0.13, r 2 = 0.93). The SDs of the residuals were 1 mL/min (VTI), 1.4 mL/min (pulmonary flow and two-dimensional), and 1.5 mL/min (M-mode).

All echocardiographic methods (M-mode, two-dimensional images, pulmonary flow) overestimated the flow probe-measured CO. Before vena cava occlusion, flow probe-measured CO was 10 ± 1 mL/min in the mice used for M-mode measurements, 11 ± 1 mL/min in the mice used for two-dimensional measurements, and 10 ± 1 mL/min in the mice used for pulmonary measurements. CO measured near-simultaneously using echocardiography was 21 ± 1.3 mL/min (M-mode), 14 ± 1 mL/min (two-dimensional images), and 17 ± 0.8 mL/min (pulmonary flow). When the measures before and during caval occlusion were analyzed, the overestimation of the flow probe-measured CO remained greatest for the M-mode (1.3 ± 0.8-fold, P < . 05 compared with the two other methods, Figure 2 A). Two-dimensional images-calculated CO overestimated flow probe-measured CO to a lesser extent than the other echocardiographic methods (overestimation of 0.3 ± 0.5-fold, P < . 05 compared with the two other methods, Figure 2 B). CO estimated from the pulmonary flow overestimated flow probe-measured CO by 0.8 ± 0.5-fold ( Figure 2 C).
Intraobserver and Interobserver Variabilities
Intraobserver and interobserver variabilities of the echocardiographic measurements are reported in Table 1 . The intraobserver variabilities were not statistically different between methods. Of note, the intra- and interobserver variabilities of the pulmonary artery diameter (expressed as the SD of the % error) were 7%. The interobserver variability of the CO derived from the pulmonary VTI was lower than that of all other methods.
Algorithm used to measure CO | Intraobserver variability of CO measurement | Interobserver variability of CO measurement |
---|---|---|
M-mode | −0.5 ± 1.22 (−1.9 ± 6.6) | 0.2 ± 1.8 (0.7 ± 10.2) |
Two-dimensional | −0.1 ± 1.0 (2.3 ± 9.4) | −0.5 ± 1.5 (−8.9 ± 14.5) |
Pulmonary flow | 0.2 ± 1.1 (1.5 ± 8.4) | −0.9 ± 1.5 (−6.1 ± 10.2) |
VTI | 0.0 ± 0.14 (−0.7 ± 4.7) | 0.0 ± 0.17 (−0.9 ± 6.6) |
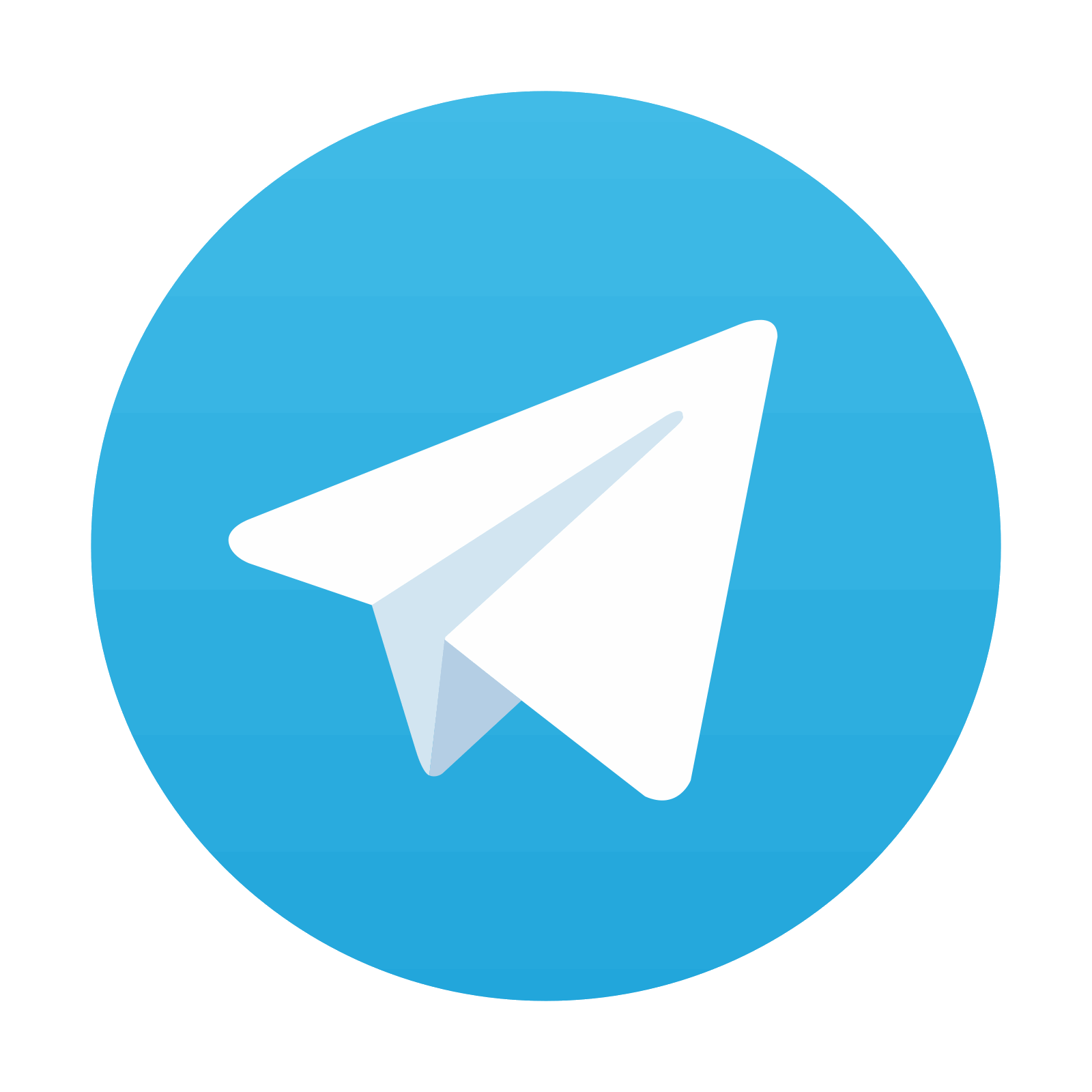
Stay updated, free articles. Join our Telegram channel
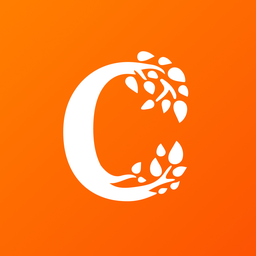
Full access? Get Clinical Tree
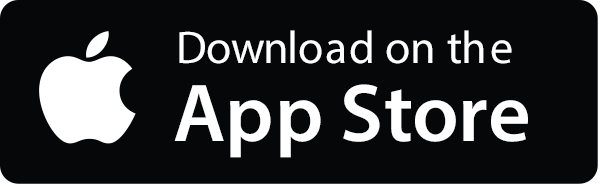
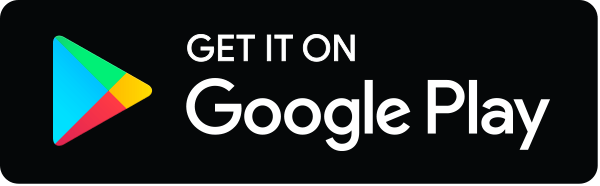
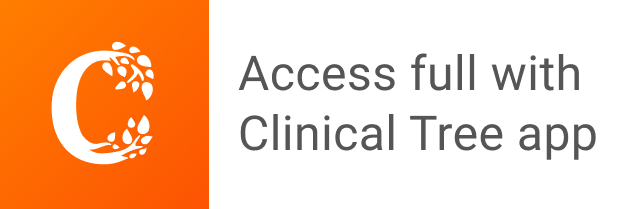