The early diastolic transmitral velocity/tissue Doppler imaging mitral annular early diastolic velocity (E/e′) ratio is used to estimate left ventricular (LV) filling pressures at rest. However, there are only limited data that validate its use during exercise. Accordingly, the aim of this study was to test the ability of E/e′ to estimate pulmonary capillary wedge pressure (PCWP) during symptom-limited exercise in patients with LV systolic dysfunction. Forty patients with severe LV dysfunction and heart failure symptoms (54 ± 12 years, 28 men) underwent simultaneous Doppler assessment of E/e′ and right-sided cardiac catheterization at rest and during a symptom-limited exercise test, at steady state levels of 30%, 60%, and 90% of their maximal exercise capacity. During exercise, all 40 patients successfully completed stage 1, yielding 40 pairs of data for comparison. Eighteen patients also successfully completed stage 2, and 5 patients also made it through stage 3, yielding 23 additional data pairs. In total, there were thus 63 pairs of data available during exercise. With exercise, heart rate increased from 77 ± 14 to 112 ± 21 beats/min. Septal E/e′ at rest correlated well with PCWP at rest (r = 0.75, p <0.01). PCWP at rest also correlated with resting mitral deceleration time (r = 0.32, p <0.01) and with the transmitral E/A ratio (r = 0.74, p <0.01). During exercise, the correlation between septal E/e′ and PCWP was weaker (r = 0.57, p <0.01) and was shifted to the right. This rightward shift was observed in patients with both separated or merged E and A velocities. In conclusion, in patients with severe LV dysfunction, although E/e′ allows accurate estimation of PCWP at rest, it appears less reliable for estimating LV filing pressure during exercise.
In patients with exertional dyspnea who have normal hemodynamics at rest, assessment of left ventricular (LV) filling pressures during dynamic exercise can be useful to establish the diagnosis of heart failure and to evaluate its severity. Pulmonary capillary wedge pressure (PCWP) measurement by right-sided cardiac catheterization is usually considered as the gold standard for assessing exercise hemodynamics in these patients. Regrettably, it is an invasive procedure that cannot therefore be applied for screening or serve as a guide to therapy in every patient with congestive heart failure. Previous studies have shown that LV filling pressures at rest can be estimated noninvasively using Doppler echocardiographic methods. In particular, the ratio of early diastolic transmitral to mitral annular velocities (E/e′) was shown to correlate well with both the LV end-diastolic pressure and the PCWP. Although it was recently suggested that this parameter can also be assessed during low-level exercise, limited data exist concerning its accuracy in estimating PCWP during maximal exercise. Accordingly, this prospective study was designed to test the ability of E/e′ to provide reliable estimates of PCWP during symptom-limited maximal exercise.
Methods
We prospectively enrolled 40 consecutive patients with LV systolic dysfunction scheduled for routine cardiac catheterization and hemodynamic evaluation. Seventeen of these patients were referred for pretransplant testing and the remaining 23 for hemodynamic evaluation of a dilated cardiomyopathy. Patients with significant aortic regurgitation, chronic obstructive pulmonary disease, anemia, or primary mitral valve disease were not considered for inclusion, as were patients with atrial fibrillation, pacemaker, and cardiac resynchronization therapy device. Each subject was informed of the investigative nature of the study, which had been approved by the Ethical Committee of our institution.
All patients performed 2 symptom-limited, multistage, dynamic bicycle exercise tests with an eBike comfort ergometer (GE Healthcare, Wisconsin). The first test was performed in the upright position and was used for determination of peak VO 2 as well as for selection of the submaximal and maximal workloads to be applied during the second test. The test was started with a workload of 10 W with increment intervals of 10 W every minute. The second test was performed a few days later in a semisupine position at steady state levels of 30%, 60%, and 90% of the maximal workload determined during the first test. During the second test, arterial and right-sided cardiac pressures, cardiac output, and all echo Doppler parameters were “simultaneously” measured at rest and during the last 3 minutes of each exercise level.
Cardiac output and right-sided cardiac hemodynamics were measured in every patient using a 7Fr Swan-Ganz catheter introduced into the pulmonary artery through the right internal jugular vein. An 18-gauge catheter was also introduced in the left brachial artery for measurement of arterial pressure and withdrawal of arterial blood samples. Cardiac output was measured by the Fick method.
Echocardiographic data were obtained with an iE33 echocardiographic system (Philips Medical Systems, Andover, Massachusetts). Images were acquired at rest in 2 positions (left lateral decubitus and semisupine) and at each stage of the exercise protocol, with the patient in the same semisupine position. Spectral Doppler recordings from the mitral inflow, and from both the septal and lateral mitral annuli, were obtained from the apical 4-chamber view to assess LV filling dynamics and mitral annular velocities, respectively. Two-dimensional and Doppler data were stored in digital format for subsequent off-line analysis. For assessment of LV diastolic filling, the pulse-wave Doppler sample volume was positioned between the tips of the mitral leaflets to derive the following variables: peak early (E) and late (A) transmitral filling velocities (cm/s) and their ratio (E/A ratio and the deceleration time of the E velocity (ms), from peak E velocity to the baseline). To ensure optimal quality of annulus velocities, gain and filters settings were adjusted to correctly visualize the onset of the e′ wave, velocity scale was adjusted as needed until 15 to 20 cm/s, and the sweep speed was optimized.
The analyses were performed offline on a Xcelera R2.1 workstation (Philips Medical Systems). Echocardiographic measurements were performed according to the recommendations of the American Society of Echocardiography. LV volumes and ejection fraction were calculated using the Simpson biplane method. The septal and lateral mitral annular velocities were measured from high-quality pulse-wave tissue Doppler tracings, and the dimensionless mitral E/e′ ratio was calculated for each annular location. In our study, merged E and A velocity referred to complete fusion of the E- and A-wave velocity. In case of partially fused mitral E and A waves, only the E/A ratio was measured. In summary, all E/e′ were calculated with either simultaneously not merged or merged E and e′.
All analyses were performed using the NCSS statistical software. Data are expressed as mean values ± 1 SD. Each M-mode and Doppler data point represents the average of 3 to 5 consecutive beats, and each PCWP value represents the average of 10 consecutive beats. Comparison among the different exercise stages was performed using a paired student t test. A p value of <0.05 was considered indicative of a statistically significant difference. The relation between hemodynamic and Doppler parameters was tested by linear regression analysis and the Pearson correlation coefficient. Two-way analysis of variance test was used for repeated measurement analysis. Receiver operating characteristic curves were constructed to determine optimal sensitivity and specificity for predicting PCWP >12 mm Hg with mitral E/e′ ratio.
Intra- and inter-observer reproducibilities were assessed in 15 randomly selected patients. The analysis was performed by 2 experienced cardiologists on the same set of images that was collected during protocol study. Variability was expressed as the mean percent error, derived as the absolute difference between the 2 sets of observations, divided by the mean of the observations.
Results
Baseline characteristics of the study patients enrolled are summarized in Table 1 . On average, the patients exercised for 9 ± 4 minutes (range 3 to 18). The peak VO 2 achieved during the first exercise averaged 14 ± 5 ml kg −1 min −1 (range 7 to 24 ml kg −1 min −1 ), corresponding to 46 ± 15% of the maximal age- and gender-predicted peak VO 2 (range 23% to 81%). Dyspnea rather than leg fatigue was the most frequent cause of exercise cessation (58%). Hemodynamic changes during the second exercise test are summarized in Table 2 . With dynamic exercise, heart rate, mean blood pressure, cardiac index, mean pulmonary pressure, PCWP, and right atrial pressure all increased significantly.
Variable | Value |
---|---|
Age (years) | 54 ± 12 |
Men/women | 28/12 |
Body mass index (kg/m²) | 26.4 ± 4.4 |
Body surface area (m²) | 1.85 ± 0.18 |
Systolic blood pressure (mm Hg) | 112 ± 25 |
Diastolic blood pressure (mm Hg) | 62 ± 9 |
Heart rate (bpm) | 77 ± 14 |
Cardiac output (L/min) | 4.39 ± 1.34 |
LV end-diastolic volume (mL/m²) | 120 ± 47 |
LV end-systolic volume (mL/m²) | 89 ± 45 |
LV ejection fraction (%) | 27 ± 11 |
NYHA class III | 29 (73%) |
Ischemic cardiomyopathy | 14 (35%) |
Maximal exercise workload (watts) | 76 ± 31 |
VO 2max (mL/kg/min) | 13.9 ± 4.5 |
Mean hemoglobin level (gr/dl) | 13.1 ± 1,5 |
Medication | |
ARB/ACEI | 40 (100%) |
β-blockers | 36 (90%) |
Calcium-channel blockers | 1 (3%) |
Digoxin | 2 (5%) |
Loop diuretics | 32 (80%) |
Aldosterone antagonists | 34 (85%) |
Parameters | Rest | Exercise | p Value |
---|---|---|---|
Heart rate (bpm) | 77 ± 14 | 112 ± 21 | < 0.001 |
Systolic blood pressure (mm Hg) | 112 ± 25 | 150 ± 36 | < 0.001 |
Diastolic blood pressure (mm Hg) | 62 ± 9 | 73 ± 13 | < 0.001 |
Mean blood pressure (mm Hg) | 80 ± 15 | 99 ± 21 | < 0.001 |
Mean pulmonary artery pressure (mm Hg) | 27 ± 8 | 45 ± 12 | < 0.001 |
Right atrial pressure (mm Hg) | 5 ± 4 | 10 ± 6 | < 0.001 |
PCWP (mm Hg) | 15 ± 7 | 24 ± 9 | < 0.001 |
Cardiac output (L/min) | 4.39 ± 1.34 | 7.29 ± 2.64 | < 0.001 |
Cardiac index (L/min/m 2 ) | 2.4 ± 0.7 | 4.0 ± 1.4 | < 0.001 |
E (cm/s) | 72 ± 19 | 103 ± 28 | < 0.001 |
A (cm/s) | 55 ± 24 | 61 ± 27 | = 0.013 |
E/A | 1.59 ± 0.93 | 1.76 ± 1.03 | = 0.565 |
E deceleration time (ms) | 125 ± 52 | 100 ± 20 | = 0.021 |
Septal e′ (cm/s) | 4.8 ± 2.4 | 7.8 ± 3.6 | < 0.001 |
Septal E/e′ | 17.7 ± 7.6 | 15.5 ± 6.6 | = 0.017 |
Lateral e′ (cm/s) | 5.9 ± 2.6 | 10.4 ± 3.4 | < 0.001 |
Lateral E/e′ | 14.3 ± 7.4 | 10.8 ± 4.1 | < 0.001 |
Average e′ | 5.4 ± 1.8 | 9.1 ± 3.2 | < 0.001 |
Average E/e′ | 14.5 ± 5.3 | 12.3 ± 4.1 | < 0.001 |
At rest, hemodynamic and echocardiographic data were acquired both in left lateral decubitus and semisupine positions, giving 80 pairs of data for comparison. All tissue Doppler imaging measurements were obtained successfully. Image quality was good in 98% of patients. An E/e′ at rest of <8 was seen in 7 measurements. The mean PCWP was 7 ± 3 mm Hg in this group. Mean PCWP was 10 ± 5 mm Hg in the 28 measurements with an E/e′ at rest from 8 to 15 and 20 ± 7 mm Hg in the 45 measurements with an E/e′ at rest of >15 (p <0.01 for difference between groups). As shown in Figure 1 , there was a correlation between septal E/e′ and mean PCWP at rest (r = 0.75, p <0.01). Bland-Altman analysis ( Figure 1 ) revealed a mean difference of 2.4 ± 5.8 mm Hg between Doppler and catheter wedge pressure. This correlation remains good with patients without merged E and A velocities (r = 0.75, p <0.01; Figure 1 ). In contrast, no correlation was found between lateral E/e′ and PCWP (r = 0.14, p = ns). Correlation between average E/e′ and PCWP was weak (r = 0.44, p = ns). Using receiver operating characteristic curve analysis, a septal E/e′ of >15 at rest had a sensitivity of 91% and a specificity of 77% for identification of an elevated PCWP at rest (>12 mm Hg). PCWP at rest also correlated with mitral deceleration time at rest (r = 0.32, p <0.01) and with the transmitral E/A ratio (r = 0.74, p <0.01).
All patients successfully completed stage 1 of the second exercise test. Eighteen patients also completed stage 2, of which 5 also made it through stage 3, yielding a total of 63 pairs of data for comparison. During the 3 different stages of exercise, all tissue Doppler imaging measurements were obtained successfully, and image quality was considered to be good in 90% of patients. In the 20 patients with a normal mean PCWP at rest (<12 mm Hg), PCWP remained normal during exercise in 10 and became elevated in the remaining 10 patients. In the 10 patients with a normal mean PCWP both at rest and with exercise, displayed E/e′ was low at rest (9.2 ± 4.1) and did not change significantly with exercise (8.8 ± 2.4, p = 0.509). In the 10 patients in whom PCWP increased with exercise, E/e′ did not change from rest to exercise (from 12.8 ± 4.6 to 11.9 ± 4.1, p = 0.593). Finally, in the 20 patients with an elevated mean PCWP at rest, E/e′ was elevated at rest (22.5 ± 6.5) and did not change with exercise (19.5 ± 6.1, p = 0.119). As shown in Figure 2 , a weaker correlation between septal E/e′ and mean PCWP was observed during exercise (r = 0.57, p <0.01). Bland-Altman analysis ( Figure 2 ) revealed a mean difference of −8.7 ± 8.2 mm Hg between Doppler and catheter wedge pressure. A significant, albeit weaker, correlation was also found between lateral E/e′ and PCWP (r = 0.40, p <0.01) or between average E/e′ and PCWP (r = 0.52, p <0.01). A weak correlation was found between PCWP and the mitral deceleration time (r = 0.08, p <0.01). However, a significant correlation between the transmitral E/A ratio and PCWP (r = 0.70, p <0.01) was found when E and A waves were not yet merged. Moreover, the relation between septal E/e′ and PCWP during exercise in these patients presenting nonmerged E and A velocities was better (r = 0.72) than in the entire cohort ( Figure 2 ). Moreover, we observed that PCWP increased with increasing heart rate and exercise intensity ( Figure 3 ), whereas the septal E/e′ did not and even tended to decrease ( Figure 4 ).
Figure 5 shows the individual changes in septal E/e′ and PCWP from rest to peak exercise. As illustrated, exercise resulted in a rightward shift of the relation between E/e′ and PCWP. To gain further insight into the mechanisms underlying the rightward shift of the relation between E/e′ and PCWP, we analyzed separately the exercise-induced changes in E, e′, E/e′, and PCWP in patients with and without merging of early and late filling or annular velocities. Indeed, with increasing heart rates, the E (or e′) and A (or a′) velocities were frequently merged. The percentage of patients with merged E and A velocities was 18% at rest and increased with the intensity of exercise: 40% at stage 1, 75% at stage 2, and 80% at stage 3. Otherwise, despite a better relation between septal E/e′ and PCWP during exercise in patients presenting nonmerged E and A velocities ( Figure 2 ), the individual changes between rest and peak exercise remained unpredictable ( Figure 5 ).
As listed in Table 3 , exercise similarly increased PCWP in patients with or without merged E and A velocities. Exercise also increased E in both groups, albeit significantly more so in patients with merged E and A velocities. In contrast, e′ did not change with exercise in patients without merged E and A velocities, whereas it significantly increased in patients with merged E and A velocities. Accordingly, E/e′ decreased significantly in patients with merged E and A velocities but remained unchanged in patients without merging of early and late velocities.
Parameters | Patients With Separated E and A Waves | Patients With Merged E and A Waves | ||
---|---|---|---|---|
Rest | Exercise | Rest | Exercise | |
Heart rate (bpm) | 70 ± 14 | 101 ± 19 | 81 ± 13 | 120 ± 18 |
E (cm/s) | 69 ± 18 | 91 ± 22 | 74 ± 20 | 111 ± 29 |
A (cm/s) | 51 ± 22 | 61 ± 27 | 59 ± 25 | NA |
E/A | 1.69 ± 0.99 | 1.76 ± 1.03 | 1.49 ± 0.89 | NA |
e′ septal (cm/s) | 4 ± 1 | 5 ± 2 | 5 ± 3 | 9 ± 4 |
e′ lateral (cm/s) | 6 ± 3 | 9 ± 3 | 6 ± 2 | 11 ± 4 |
Average e′ (cm/s) | 5 ± 2 | 8 ± 2 | 6 ± 2 | 10 ± 3 |
E/e′ septal | 19 ± 8 | 18 ± 7 | 17 ± 7 | 15 ± 6 |
E/e′ lateral | 15 ± 8 | 11 ± 4 | 14 ± 7 | 11 ± 4 |
Average E/e′ | 15 ± 6 | 12 ± 4 | 14 ± 5 | 12 ± 4 |
E deceleration time (ms) | 136 ± 62 | 117 ± 13 | 118 ± 15 | – |
PCWP (mm Hg) | 15 ± 8 | 23 ± 10 | 15 ± 7 | 25 ± 9 |
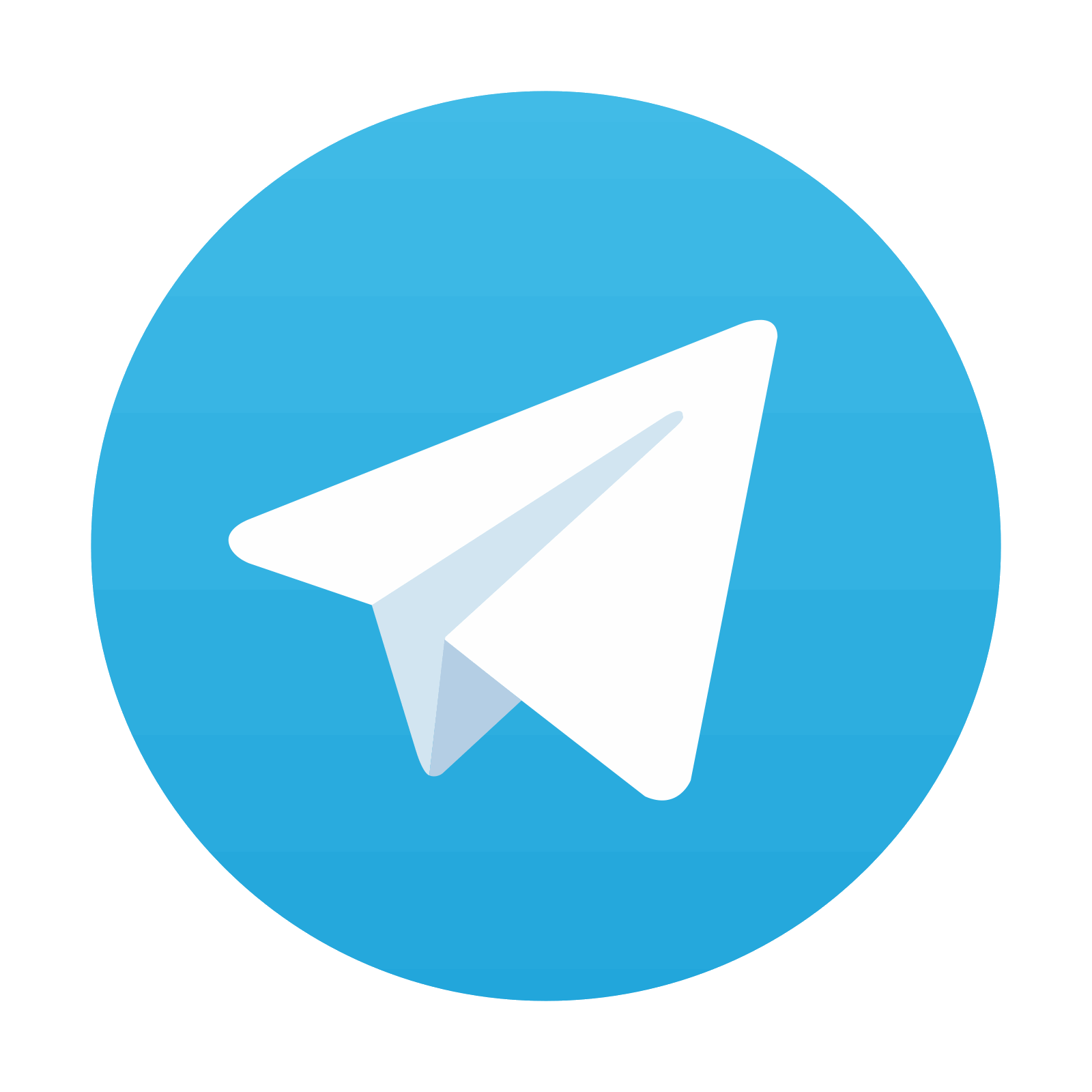
Stay updated, free articles. Join our Telegram channel
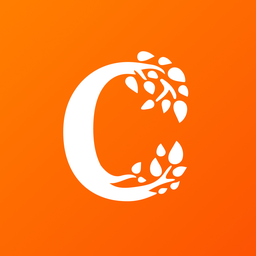
Full access? Get Clinical Tree
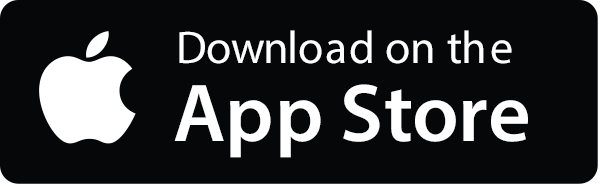
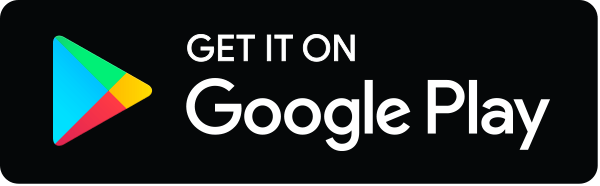
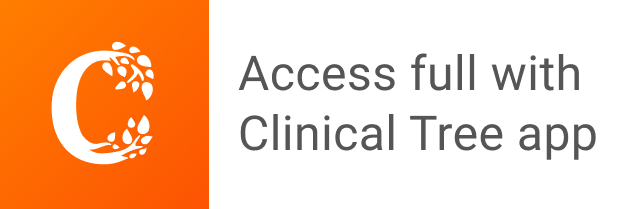