Name
Target
Category
[13N]NH3
Perfusion
Flow tracer
[15O]-water
Perfusion
Flow tracer
[18F]-flurpiridaz
Perfusion
Enzyme inhibitor
[82Rb]-rubidiumchloride
Perfusion
Flow tracer
[11C]-CGP-12177
Beta-adrenoceptor
Receptor antagonist
[11C]-CGP-12388
Beta-adrenoceptor
Receptor antagonist
[11C]-MQNB
Muscarinic receptor
Receptor antagonist
[11C]-mHED
Presynaptic NE transport
Transporter substrate
[11C]-palmitate
Fatty acid metabolism
Enzyme substrate
[11C]-acetate
Oxidative metabolism
Enzyme substrate
7.2.1.2 Tracer Development Toward Clinical Use
To develop a tracer towards clinical use, often many derivatives of a compound are involved, especially for receptor-targeted tracers, but also for substrate-based tracers. When a lead compound is selected in silico, the compound and some of its nearly related derivates are tested first in vitro and thereafter in vivo using appropriate animal models. When the proof of concept is established, both by binding studies using, e.g., blockers to proof that the tracers reaches the intended target, as well as by in vivo studies to show that imaging using the new potential radiopharmaceutical is feasible (Elsinga and Dierckx 2014). When both goals are achieved, the tracer can be developed further as a radiopharmaceutical (see Sect. 7.2.6 and onwards). Several animal models can be used for investigation of, e.g., receptor binding properties, as well as perfusion imaging. First, the Langendorf model, exploring a rodent heart for ex vivo perfusion as well as receptor binding/transporter substrate effects can be used for initial suitability testing of novel tracers (Hillman et al. 2014). Second, when ex vivo heart models like these demonstrate the feasibility of the investigated tracer, more complex and sophisticated models can be used for a pilot study, e.g., investigating effects on perfusion and/or innervation using the same tracers, eventually using both a perfusion as well as an innervation tracer. Suitable animal models are mimicking heart failure by induction of blood pressure overload, as caused by aortic constriction in, for instance, murine animal models (Mühlfeld et al. 2013).
7.2.2 Cardiac Innervation Imaging: An Overview on Currently Available Radiopharmaceuticals
The cardiac nervous system in humans is innervated by sympathetic and parasympathetic nerve systems. The main neurotransmitters responsible for this innervation are norepinephrine and acetylcholine. It is known that alterations of this innervation system can lead to various heart diseases, such as arrhythmias and heart failure (Bengel and Schwaiger 2004). Therefore, visualizing innervation of the heart may be an option for diagnosing heart diseases in an early stage. Available tracers for the sympathetic neurons can be divided into two groups, radiolabeled catecholamines and radiolabeled catecholamine analogues. The latter group is resistant to biotransformation by monoamine oxidase (MAO) and catechol-O-methyltransferase (COMT). Differences between the available tracers include their affinity and specificity for uptake-1 (a transport system allowing reuptake into nerve terminals) and the storage of the tracer in vesicles. A limited number of tracers, e.g., [123I]-N-methyl-4-iododexetimide, are available for the mapping of parasympathetic neurons, because of the low density of these neurons in the myocardium and the high specificity for acetylcholine in the uptake and storage system (Bengel and Schwaiger 2004; Langer and Halldin 2002; Kassiou et al. 1996).
7.2.3 [123I]-metaiodobenzylguanidine
The most frequently used radiopharmaceutical for visualization of the sympathetic system is [123I]-metaiodobenzylguanidine ([123I]-MIBG), in combination with single-photon emission computed tomography (SPECT) imaging. By processing the patient images obtained after injection of the tracer, it is possible to calculate a heart-to-mediastinum ratio, which is used as an estimation of the uptake of catecholamine throughout the heart (Boersma et al. 2002; Weiland et al. 2010). In addition, the sympathetic tonus can be reflected by measuring the ratio of cardiac uptake between early and delayed images. The latter is also called the washout rate (Bengel and Schwaiger 2004; Bengel 2011). The washout rate and the heart-to-mediastinum ratio indicate norepinephrine release and uptake from and into the nerve terminal. Therefore, [123I]-MIBG is a commonly used tracer for the assessment of sympathetic innervation of the heart in heart failure (Tamaki and Yoshinaga 2011).
7.2.4 [11C]-metahydroxyephedrine
The most frequently used tracer when mapping sympathetic neurons with positron emission tomography (PET) is carbon-11-metahydroxyephedrine (mHED). mHED is a catecholamine analogue and therefore not metabolized by MAO or COMT. It can be produced with sufficiently high specific activity to avoid measurable physiologic effects, and it has low nonspecific binding. When reuptake of the neurotransmitters into the nerve terminals in isolated perfused rat hearts is blocked with desipramine, mHED shows an accelerated washout, indicating that the norepinephrine transporter is responsible for this reuptake. This is also proven by the accelerated washout of mHED when norepinephrine is added to the perfusate. Because of the increased washout in these situations, it is believed that mHED is released from and transported back into sympathetic neurons continually. In contrast with [123I]-MIBG, which has lower uptake in the inferior myocardium, mHED has homogeneous high uptake in the left ventricle throughout all myocardial segments (Bengel and Schwaiger 2004).
7.2.5 Perfusion and Viability Tracers
Perfusion tracers are used to depict myocardial blood flow and are a tool to determine myocardial cell viability. Myocardial perfusion imaging tracers are regularly radiolabeled molecules which are (partially) entrapped within the cardiomyocyte (Candell-Riera et al. 2009; Di Carli et al. 2007). With this nuclear medicine procedure, it is possible to illustrate the function of the heart muscle. This enables the evaluation of several heart conditions from coronary artery disease (CAD) to hypertrophic cardiomyopathy and abnormalities in the motion (using gated PET) of the myocardial wall.
PET myocardial perfusion imaging (MPI) is a very accurate technique in making prognoses in patients with suspected or known CAD. MPI has an average sensitivity and specificity of around 90 % for detection of angiographically significant CAD. PET MPI has proven to be useful as the initial test for detection as well as the extent and location of myocardial ischemia. Imaging with SPECT and PET can be used for detecting obstructive CAD and/or to estimate viability of the myocard. Identifying ischemia in patients with heart failure (HF) is an important step for evaluation and renders prognostic information. According to Canadian HF guidelines, it is recommended to use radionuclide perfusion imaging, SPECT most commonly, for evaluating the presence of infarction, ischemia, and/or viability. The most commonly used and available SPECT tracers are [201Tl], [99mTc]-tetrofosmin, and [99mTc]-sestamibi (Paterson et al. 2013).
Comparing PET with SPECT, PET imaging has shown to be a more comprehensive functional and anatomical assessment of the cardiovascular system. For instance, PET has better spatial resolution, higher sensitivity, and ability to measure distribution of tracers in absolute terms as a function of time (Anagnostopoulos et al. 2013).
The most common positron-emitting radiopharmaceuticals for cardiac perfusion imaging are rubidium-82 [82Rb], [13N]NH3, and oxygen-labeled water ([15O]-water). Among these tracers, the most widely used in clinical practice are [82Rb] and [13N]NH3 (Anagnostopoulos et al. 2013).
All three PET tracers have short half-lives (<10 min), making it possible to repeatedly measure at stress and rest. Another major property of current perfusion tracers is they have a high first-pass extraction fraction at different flow rates. Lower extraction occurs at high flow rates, leading to a decrease in accuracy of ischemia detection, representing an error source of underestimation (Rischpler et al. 2012). PET imaging is able to quantify myocardial blood flow and flow reserve. This results in better assessment of the functional importance of CAD in HF. Diagnostic accuracy for PET to detect significant CAD is much higher than SPECT (sensitivity 91 % and specificity 91 %). [18F]-FDG PET imaging is considered one of the gold standards for viability imaging. It has a high accuracy for predicting functional recovery, with a sensitivity of 85–90% (Paterson et al. 2013).
7.2.6 Product Development
Good manufacturing practice (GMP) comprises a defined set of regulations, defining all requirements needed for the correct development and manufacture of a medicinal product under adequately controlled circumstances. According to GMP, new medicinal products need to be thoroughly tested, evaluated and released before it is allowed to administer them to humans (EU-GMP-website).
In most cases, such new medicinal product is classified as an investigational medicinal product (IMP) and is tested during a controlled clinical trial. Then, an ethics committee is involved to review and approve the clinical trial procedure and to ensure the safety of the participating subjects (see Sect. 7.4).
When designing a new radiopharmaceutical, first of all the chemical synthesis of the compound needs to be robust, safe, and reproducible. Furthermore, there is a need to have an accurate quality control (QC) method to warrant correct QC results and thus appropriate release of the tracer before administration (EU-GMP-website, annex 13).
Moreover, the produced radiochemical should be transformed into a radiopharmaceutical. This process is called formulation. Usually, formulation is executed by dilution of the radiochemical dissolved in, for instance, ethanol using water for injections. This, and all requirements sustaining good quality of the radiopharmaceutical, will be discussed in the upcoming paragraphs.
7.3 Step: What Is Needed to Ensure the Clinical Application of a Tracer?
7.3.1 Animal Toxicity Testing
In order to study the toxicity of a newly developed radiopharmaceutical, the biodistribution of the tracer in animals (rodents and non-rodents) has to be investigated. To ensure the efficacious performance of animal experiments, a risk assessment can help to determine the extent of animal studies needed. The biodistribution studies include measurement of plasma levels of the tracer as well as its uptake in different tissues, such as the kidneys or the liver, and the elimination route. Also, the stability of the tracer in the animal plasma has to be determined. Furthermore, it should be investigated which metabolites are formed as well as their excretion via the kidneys and/or gut.
In order to study the acute toxicity of the tracer, it has to be administered in single intravenous doses at different concentrations on day 1 and followed by observation of the animals for 7 days. During this period, it is studied whether there are any clinical signs that might point to toxicity such as changes in body weight, respiratory function, food consumption, eye toxicity, and after termination of the animal: clinical pathology, organ weight, and/or macroscopic/microscopic organ picture. These tests result in the determination of a no-adverse-effect level (NOAEL) and a maximum tolerated dose (MTD). For low-dose radiopharmaceuticals (<100 μg of tested substance in human volunteers), a microdosing approach can be used for toxicity testing, which comprises of single dose toxicity testing at a 1,000-fold dose (ICH M3-website).
7.3.2 Introduction into Good Manufacturing Practice (GMP)
GMP is a quality assurance system which is applied in the pharmaceutical industry. Quality assurance is applied because it is impossible to analyze every (bio)chemical or physical aspect of all pharmaceutical products used before release of a tracer. Because the product quality is the core issue for pharmaceuticals to ensure their efficacy and safety, GMP should be used at every stage of the product life cycle: from the manufacturing of investigational medicinal products, technology transfer, commercial manufacturing to product discontinuation. For pharmaceutical products manufactured by the holder of a manufacturing license, it must be ensured they are fit to their intended use. Authorization for manufacturing is required for all pharmaceutical manufacturers in the European Union, whether the products are sold or used within or outside of the Union, or not.
The final pharmaceutical product must also comply with the requirements of a marketing authorization or an investigational medicinal product dossier (IMPD), and, when applicable, a clinical trial authorization, and does not place patients at an unexpected risk due to inadequate quality or efficacy (EU-GMP-website).
As stated above, GMP ensures that products are consistently produced and controlled to the quality standards appropriate to their intended use and as required by the marketing authorization (MA), clinical trial authorization, or product specification. Both production and quality control are part of GMP. EMA (European Medicine Agency) and FDA (Food and Drug Administration) are the respective regulators in the EU and the USA. These bodies ensure the quality maintenance of all licensed pharmaceuticals. Furthermore, the FDA serves as a pharmaceutical inspectorate as well. Within the EU, inspectors are organized at a national level for each member state.
The basic requirements of GMP are as described below:
1.
All manufacturing processes are clearly defined, systematically reviewed in the light of experience and shown to be capable of consistently manufacturing medicinal products of the required quality and complying with their specifications.
2.
All necessary facilities for GMP are provided, including:
Appropriately qualified and trained personnel
Adequate premises and space
Suitable equipment and services
Correct materials, containers, and labels
Approved procedures and instructions, in accordance with the pharmaceutical quality system
Suitable storage and transport
3.
Instructions and procedures are written in an instructional form in clear and unambiguous language, specifically applicable to the facilities provided.
4.
Procedures are carried out correctly, and operators are trained to do so.
5.
Records are made, manually and/or by recording instruments, throughout the manufacturing process, to demonstrate that all the steps required by the defined procedures and instructions were in fact taken and that the quantity and quality of the product was as expected.
6.
Every change in critical processes is recorded and appropriately validated according to an authorized change control procedure.
7.
Any significant deviations are fully recorded, investigated with the objective of determining the root cause and appropriate corrective and preventive action implemented.
8.
Records of manufacture, including distribution (which enables to trace the complete history of a batch), are retained in a comprehensible and accessible form.
9.
The distribution of the products minimizes any risk to their quality and is performed in accordance with the guidelines concerning good distribution practice (GDP).
10.
A system is available to recall any batch of product, from sale or supply.
11.
Complaints about products are examined, the causes of quality defects investigated, and appropriate measures taken in respect of the defective products and to prevent reoccurrence (EU-GMP-website).
7.3.2.1 GMP Essentials for Radiopharmaceuticals
The preparation and use of radiopharmaceuticals are regulated by defined EU or FDA guidelines and rules. Within the EU-GMP, annex 3 defines how production of radiopharmaceuticals should take place. Guidelines, such as the EU-GMP intended for the production of medicinal products, should also be used, but it must be taken into consideration that some regulations do not cover special characteristics of radiopharmaceuticals, such as:
Short shelf life, due to short physical half-life radionuclide
Small-scale preparations; these are defined as preparations resulting in <25 patient doses. This is typical for radiopharmaceutical production in a hospital
Low or absent toxicity of final product, due to particular no-carrier-added nature of radiopharmaceuticals or a low dose to be administered to each patient
Nuclear energy laws regulating some aspects of production requirements, such as protection of the involved staff, which are not covered by GMP
For the production of radiopharmaceuticals, the “guidelines on good radiopharmacy practice (GRPP)” and “guidelines on current good radiopharmacy practice (cGRPP) for the small-scale preparation of radiopharmaceuticals” were developed. These can be used for small-scale preparations at nonindustrial sites such as hospital pharmacies, nuclear medicine departments, and PET centers (Elsinga et al. 2010). Notwithstanding the application of cGRPP, the guidance of, for instance, of EU-GMP annex 3 on radiopharmaceutical production and annex 1 on sterile manufacturing, is currently regarded as mandatory in EU member states. Although national inspectorates will have different interpretations of these rules in their respective countries, most countries in the western world as well as many other states regard radiopharmaceuticals as regular medicinal products up until now (EU-GMP-website). However, in April 2014, the European Parliament has accepted new regulations towards production of radiopharmaceuticals (Anonymous 2014, Publication of the EU-parliament). These will be operational from 2015 onwards. One very important aspect is that for clinical trial use within a specific member state, a GMP license is no longer required. This may implicate that cGRPP will become more important as standard guidance for radiopharmaceutical production within the EU. Nevertheless, this does not mean that the quality of radiopharmaceutical products for clinical trial use should be less well defined after 2015. Furthermore, the policies of inspectorates upon licensed and clinically used radiopharmaceuticals are expected to remain unchanged.
In the USA, the situation is different compared to the EU. As of December 2011, the US legislation on production of radiopharmaceuticals has been amended. In the current situation, each production site for radiopharmaceuticals needs to have a limited license (abbreviated new drug application) for each PET radiopharmaceutical. Furthermore, GMP regulations on PET drugs are given in Chapter <823 > of the 32nd Edition of the US Pharmacopeia. Other radiopharmaceuticals need to be licensed or defined as new chemical entity to be part of a clinical trial. However, the FDA recognizes that many PET tracers have no commercial value and therefore enforces less strict rules compared to otherwise classified pharmaceuticals (FDA-Guidance PET Drugs 2011).
Moreover, when using new radiopharmaceuticals in clinical trials, cGRPP, and the guidelines on good clinical practice should be followed in addition to GMP, and cGRPP guidelines have a main focus on small-scale radiopharmaceuticals (SSRP). In contrast to GMP, cGRPP also applies for the labeling procedures of licensed kit radiopharmaceuticals.
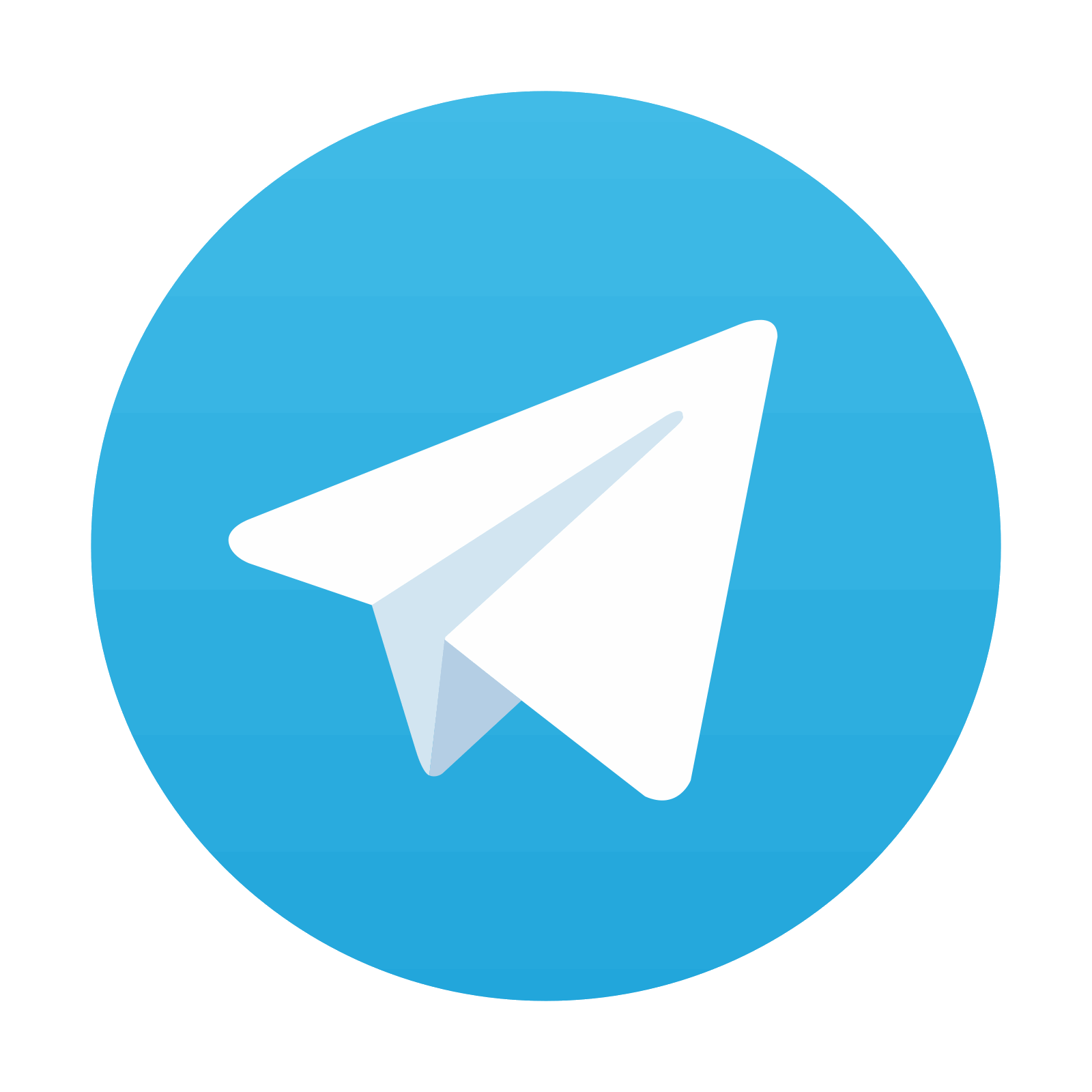
Stay updated, free articles. Join our Telegram channel
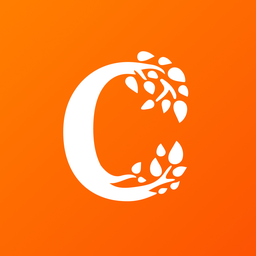
Full access? Get Clinical Tree
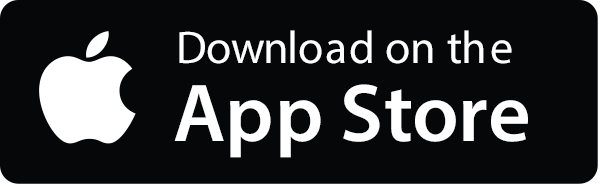
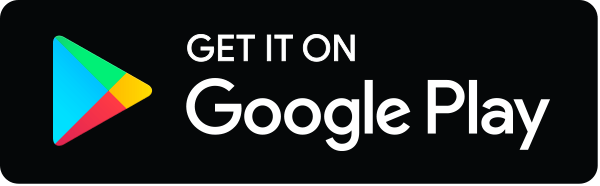