© Springer-Verlag Berlin Heidelberg 2015
Riemer H.J.A. Slart, René A. Tio, Philip H. Elsinga and Markus Schwaiger (eds.)Autonomic Innervation of the Heart10.1007/978-3-662-45074-1_33. Development of Heart Failure and the Role of the Autonomic Nervous System of the Heart
(1)
Department of internal medicine, Ghent University, Ghent, Belgium
3.1 Introduction
3.3.1 Pathophysiology
3.3.2 Effects During Exercise
3.3.3 Therapeutic Implications
3.4.1 Pathophysiology
3.4.2 Effects During Exercise
3.4.3 Therapeutic Implications
Abstract
Heart failure is a clinical syndrome that develops in response of a cardiac insult, resulting in a decline of cardiac performance. Several neurohormonal mechanisms are activated in response to the underlying myocardial dysfunction, including the autonomic nervous system. Patients with heart failure are characterized by an abnormally activated sympathetic and altered parasympathetic tone, with also attenuated cardiovascular reflexes and a maladaptive downregulation of adrenergic nerve terminals. During exercise, an inappropriate rise in ventilation occurs as well as an enhanced peripheral vasoconstriction in order to preserve an adequate blood pressure level. Although the activation of these systems can initially compensate for the depressed myocardial function, their long-term activation results in a further impairment of cardiac function leading to progression of heart failure with fatigue and dyspnea being major barriers for exercise tolerance. Medication, in particular beta-blocker and ACE-inhibitor therapy, influences both the sympathetic and parasympathetic tone. The sympathetic tone may also be modulated by cardiac resynchronization therapy whereas vagus nerve stimulation may increase the parasympathetic activity. Through its impact on the periphery, physical exercise is also able to influence both the sympathetic and parasympathetic nervous system..
Abbreviations
[123I]-MIBG
Iodine-123 metaiodobenzylguanidine
ANS
Autonomic nervous system
CNS
Central nervous system
CO
Cardiac output
CRT
Cardiac resynchronization therapy
nNOS
Neuronal nitric oxide synthase
NO
Nitric oxide
PSNS
Parasympathetic nervous system
RAAS
Renin-angiotensin-aldosterone system
SNS
Sympathetic nervous system
3.1 Introduction
Heart failure is a clinical syndrome that develops in response of a cardiac insult, resulting in a decline of cardiac performance. Several neurohormonal mechanisms are activated in response to the underlying myocardial dysfunction, including the sympathetic nervous system (SNS) and the renin-angiotensin-aldosterone system axis (RAAS axis). Although the activation of these systems can initially compensate for the depressed myocardial function, their long-term activation results in a further impairment of cardiac function leading to progression of heart failure and cardiac decompensation (Mann and Bristow 2005; Triposkiadis et al. 2009).
As pointed out by Parati and Esler (2012), sympathetic activation occurs subsequently to the development of heart failure and then impacts adversely on clinical outcome. This is in contrast to essential hypertension where SNS activation is already important in the initiation and the maintenance of hypertension. Also, there is now clear evidence that besides sympathetic activation, also reduced vagal function plays a role in the development of heart failure (Bibevski and Dunlap 2011). In this chapter we will briefly review the normal cardiovascular actions of the autonomic nervous system (ANS) and then discuss the pathophysiology and potential therapeutic implications of sympathetic hyperactivity and reduced vagal function in heart failure.
3.2 The ANS and Normal Cardiac Function
In the normal heart, the SNS has different cardiovascular actions, including acceleration of the heart rate, increase in cardiac contractility, reduction of venous capacitance, and constriction of resistance vessels. The cardiac sympathetic nerve fibers are located subepicardially and travel along the major coronary arteries (Triposkiadis et al. 2009; Zipes 2008). The sympathetic outflow to the heart and peripheral circulation is regulated by cardiovascular reflexes. Afferent fibers are carried towards the central nervous system (CNS) by autonomic nerves, whereas efferent impulses travel from the CNS towards different organs. The main reflex responses originate from the aortic arch and carotid baroreceptors (SNS inhibition), cardiopulmonary baroreceptors (including the Bezold-Jarish reflex, SNS inhibition), cardiovascular low-threshold polymodal receptors (SNS activation), and peripheral chemoreceptors (SNS activation) (Malliani et al. 1983). As already summarized by Van Stee (1978), the effect of SNS activation on the periphery is mediated by 4 pathways: (1) norepinephrine-releasing neurons through the right stellate ganglion reaching the sinus and atrioventricular nodes (resulting in an increase in heart rate and shortening of atrioventricular conduction) and through the left stellate ganglion reaching the left ventricle (resulting in an increase in contractile strength), (2) epinephrine released in circulation by the adrenal cortex affecting both the myocardium and peripheral vessels, (3) direct effect on peripheral vessels through local release of epinephrine and norepinephrine, and (4) circulating norepinephrine which can act on multiple locations (e.g., increase in heart rate during exercise in heart transplant recipients). Norepinephrine and epinephrine bind to specific adrenergic receptors, of which there are at least nine subtypes (3 α1-receptor subtypes, 3 α2-receptor subtypes, and 3 β-receptor subtypes). In the human heart, activation of β1- and β2-adrenergic receptors is the most powerful physiologic mechanism to acutely increase cardiac performance and the β-adrenergic receptor density is greatest at the apical myocardium (Leineweber et al. 2002). Approximately 80 % of norepinephrine released by the sympathetic nerve terminals is recycled by the norepinephrine transporter 1, whereas the remainder clears into the circulation (Feldman et al. 2005).
The parasympathetic nervous system (PSNS) affects the cardiovascular system by slowing heart rate through vagal impulses. The parasympathetic fibers run with the vagus nerve subendocardially and are mainly present in the atrial myocardium and less abundantly in the ventricular myocardium (Triposkiadis et al. 2009; Zipes 2008). Acetylcholine released from postganglionic cardiac parasympathetic nerves reduces heart rate by binding to muscarinic cholinergic receptors (primarily M2 subtype) on sinoatrial nodal cells (Brodde et al. 2001; Katona et al. 1977). Parasympathetic-mediated changes in heart rate are initiated primarily in the CNS or originate from activation or inhibition of sensory nerves. Stimulation of arterial baroreceptors, trigeminal receptors, and subsets of cardiopulmonary receptors with vagal afferents reflexively increases cardiovagal activity and decreases heart rate. In contrast, stimulation of pulmonary stretch receptors with vagal afferents and subsets of visceral and somatic receptors with spinal afferents reflexively decrease cardiovagal activity and increase heart rate (Chapleau and Sabharwal 2011). Importantly, the PSNS and the SNS are often working interactively with opposing resulting effects.
3.3 Heart Failure and Sympathetic Hyperactivity
3.3.1 Pathophysiology
Patients with heart failure are characterized by an abnormally activated sympathetic and altered parasympathetic tone, with also attenuated cardiovascular reflexes and a maladaptive downregulation of adrenergic nerve terminals (Himura et al. 1993; Liang et al. 1989). In the early changes of heart failure, there is a selective cardiac change in the autonomic regulation with a decrease in heart rate variability and a selective increase in cardiac norepinephrine spillover in order to preserve cardiac output (CO). Chronic persistent myocardial dysfunction is associated with a more generalized sympathetic hyperactivity. Evidence for this increased sympathetic activity in patients with heart failure includes increased central sympathetic outflow and increased norepinephrine spillover to plasma from activated sympathetic nerve fibers and consequently increased plasma norepinephrine levels. Besides the increased muscle sympathetic nerve activity and norepinephrine spillover, patients with heart failure and reduced ejection fraction may also have a decreased neuronal density and a decreased neuronal function resulting in decreased norepinephrine concentration within the cardiomyocytes. Compared with myocardium of healthy individuals, the myocardium of patients with chronic left ventricular dysfunction is also characterized by a significant reduction of presynaptic norepinephrine uptake and postsynaptic β1-adrenergic receptor density (Caldwell et al. 2008; Ungerer et al. 1993). This latter phenomenon has been documented in patients after acute myocardial infarction where it contributes to adverse left ventricular remodeling, in patients with heart failure due to dilated cardiomyopathy as well as in patients with hypertrophic cardiomyopathy who develop left ventricular dilatation and heart failure (Choudhury et al. 1996; Merlet et al. 1993; Spyrou et al. 2002). The increase in norepinephrine levels apparently results in a decrease in β1-adrenergic receptor density and a β1-adrenergic receptor desensitization which appears to be a predominantly protective adaptation. The role of cardiac β2- and β3-adrenergic receptors as well as cardiac α1-adrenergic receptors in heart failure has not been fully elucidated yet (Triposkiadis et al. 2009).
3.3.2 Effects During Exercise
3.3.2.1 Hemodynamic Effects
During exercise, cardiovascular and respiratory responses are regulated by the ANS in order to provide a sufficient oxygen supply to the working muscles (Decherchi et al. 2007). The balance between parasympathetic and sympathetic nerve activity is mediated by the interaction between the central command, originating from the central motor areas, and peripheral feedback afferents, including the baroreflex, chemoreflex, and ergoreflex (Decherchi et al. 2007; O’Leary 2006). Sensory input from these cardiovascular afferents is projected to the nucleus tractus solitarius in the medulla oblongata, which plays a pivotal role in integrating and referring this information to other regions of the CNS with an impact on the sympathetic-parasympathetic outflow (Dampney et al. 2002).
Activation of the SNS during exercise normally induces a rise in heart rate and contractility resulting in an increased CO (Triposkiadis et al. 2009). Together with local metabolic vasodilatation, increased CO amplifies the blood flow to the working muscles. Local vasodilatation is, however, partially restrained by an SNS-mediated vasoconstriction in order to maintain an adequate level of blood pressure (Khan and Sinoway 2000).
Baroreceptors, located in the aortic arch and carotid sinuses (Kougias et al. 2010), and ergoreceptors, group III and IV skeletal muscle afferents (Kaufman et al. 1983) are both responsible for these hemodynamic changes. Once activated, the main function of these baroreceptors is to maintain the arterial blood pressure at an adequate level (set point) by increasing or decreasing peripheral vasoconstriction (Kougias et al. 2010). During exercise, a rapid resetting of this set point occurs (towards a higher level) in order to allow a higher arterial pressure and increased blood flow to adjust to the increased metabolic demands (Dampney et al. 2002). Importantly, in a significant number of patients, additional nonbaroreflex-mediated excitatory stimuli, including coexisting sleep apnea, myocardial ischemia, obesity, and inflammation, may elevate the set point for central sympathetic outflow or neurotransmitter release at rest and during exercise.
As metabolites accumulate during exercise and signal insufficient oxygen supply to the exercising muscles, ergoreceptors become activated and provide a rise in arterial pressure and blood flow primarily via an increased ventricular contractility and stroke volume with a resultant increase in CO (Crisafulli et al. 2003, 2006; O’Leary 2006). The effect of the ergoreflex on heart rate has been a matter of debate. Some authors suggested that the ergoreflex has only a minor influence on HR (Piepoli et al. 1995). However, when the mean arterial pressure is elevated, arterial baroreflex should decrease heart rate to lower mean arterial pressure (Nishiyasu et al. 1994). As heart rate remains unchanged, the influence of the ergoreflex on heart rate regulation is thought to be masked by counterregulation of the arterial baroreflex (Iellamo et al. 1999). During severe exercise the ability of the ergoreflex to elicit further increases in CO becomes limited. Pressor responses are then mediated via peripheral vasoconstriction but are smaller in comparison with the contribution of CO regulation (Augustyniak et al. 2001). In normal healthy subjects, ergoreflex activity is buffered by the arterial baroreflex. If this reflex is left unbuffered, then instead of a rise in CO, peripheral vasoconstriction in the active skeletal muscle is induced as a response to ergoreflex activation (Kim et al. 2005b).
Due to a chronic left ventricular dysfunction, a catabolic state is seen in heart failure with metabolic changes and chronic underperfusion of the skeletal muscle. A shift from type I to type IIb muscular fibers has been reported with a decrease in oxidative enzyme capacity and a concomitant rise in lactate and lactate dehydrogenase activity (Mancini et al. 1989; Schaufelberger et al. 1997). Skeletal muscle apoptosis has also been described in heart failure and is thought to be triggered by proinflammatory cytokines (Vescovo and Dalla Libera 2006; Vescovo et al. 2000).
This altered muscle metabolism may elicit an accumulation of metabolic byproducts which results in a chronic activation of the ergoreceptors and subsequently sympathetic hyperactivity. Whereas in normal conditions the ergoreflex increases CO, a shift towards peripheral vasoconstriction is seen in heart failure, further limiting blood flow and exacerbating skeletal muscle abnormalities and fatigue complaints (Clark et al. 1996; Hammond et al. 2000). The loss of the CO response likely reflects the impaired ability to increase ventricular function (Augustyniak et al. 2001; Hammond et al. 2000; O’Leary 2006), and the shift towards a peripheral vasoconstriction indicates a reduced baroreflex buffering in pathologic situations (Kim et al. 2005a).
3.3.2.2 Ventilatory Effects
Apart from hemodynamic changes, ergoreceptors are also thought to take part in ventilatory responses during exercise. However, the role of these receptors in modulating ventilation in healthy subjects has been questioned due to conflicting results in the literature (Piepoli et al. 1995, 1999; Scott et al. 2000). On the other hand, in patients with heart failure, an overactive ergoreflex mechanism has been demonstrated resulting in an excessive increase in ventilation and symptoms of breathlessness. A disruption of this reflex has been shown to correlate with several prognostic exercise parameters, including the peak VO2 and the ventilatory slope (Piepoli et al. 1996, 1999; Ponikowski et al. 2001b).
Chemoreceptors may also play a critical role in the increased ventilatory response in heart failure (Chua et al. 1996; Ponikowski et al. 2001 b). According to their location, they are subdivided into central medullary and peripheral carotid afferents with the former being particularly sensitive to changes in CO2 while the latter are activated in hypoxic conditions (Dampney et al. 2002). In patients with heart failure, enhanced hypoxic and central hypercapnic chemosensitivity has been described (Chua et al. 1996; Ponikowski et al. 1997, 2001b). This hypersensitivity is associated with a higher incidence of arrhythmias and Cheyne-Stokes respiration and indicates a poor prognosis (Ponikowski et al. 2001a).
When the chemoreflex and ergoreflex are combined, the response to ventilation is greater than the sum of the two responses separately, suggesting that their interaction has an additional stimulatory effect on ventilation (Lykidis et al. 2009).
In conclusion, in a generalized sympathetic state which is seen in patients with heart failure, an enhanced peripheral vasoconstriction occurs during exercise in order to preserve an adequate blood pressure level. Consequently, this limits blood flow in the exercising muscle and thereby further exacerbates muscular abnormalities. Concerning the ventilatory aspect, an inappropriate rise in ventilation is seen which is related to the complaints of breathlessness and indicates a poor prognosis. As such, sympathetic hyperactivity contributes to the downward vicious cycle characteristic for heart failure, with fatigue and dyspnea being the major barriers for exercise tolerance.
3.3.3 Therapeutic Implications
3.3.3.1 Medication
Several classes of medication can interact with the SNS and inhibit its activity in patients with heart failure. Chronic beta-blocker therapy has been extensively evaluated in patients with heart failure. Several large scale clinical trials have shown that bisoprolol, carvedilol, metoprolol succinate, and nebivolol reverse left ventricular remodeling, reduce the risk of hospitalization and improve survival in patients with chronic heart failure (McMurray et al. 2012). This protective effect of beta-blockers is multifactorial and related to several factors, including the inhibition of catecholamine cardiotoxic effects, β1-adrenergic receptor upregulation, attenuation of the RAAS-axis, subendocardial coronary flow enhancement, and restoration of the reflex control on the heart and circulation (Adamson and Gilbert 2006; Triposkiadis et al. 2009). A meta-analysis in almost 20,000 patients demonstrated that the cardiovascular risk reduction with beta-blockers in patients with systolic heart failure was predominantly due to heart rate reduction achieved by beta-blockade rather than the type of beta-blockade, the dose of the beta-blockade, the underlying course of heart failure, and many other potential confounders (McAlister et al. 2009). The RAAS axis is upregulated in heart failure, and the resulting angiotensin II and aldosterone production enhances the release and inhibits the uptake of norepinephrine at nerve endings. Because of this interaction with the SNS, a part of the beneficial effect of angiotensin-converting enzyme inhibitors and aldosterone antagonists in heart failure can probably be attributed to their effect on norepinephrine (Adamson and Gilbert 2006). However not all medications that interact with the SNS have shown beneficial effects in patients with heart failure. Prazosin, for example, inhibits the α1-receptor but causes an increase in cathecholamine levels which probably explains a worse outcome in clinical trials (Cohn et al. 1986). Also, moxonidine that acts through both α2- and imidazolidine receptors and causes a marked dose-related reduction in plasma norepinephrine was associated with an increased mortality in clinical trials (Cohn et al. 2003).
3.3.3.2 Device Therapy
Cardiac resynchronization therapy (CRT) is an effective therapy in patients with advanced heart failure and electrical/mechanical dyssynchrony. Several large outcome studies have shown that this device therapy is associated with an improvement of symptoms, quality of life, and survival (McMurray et al. 2012). Cha et al. (2011) recently reported that CRT modulates sympathetic function by upregulating presynaptic receptor function as evidenced by increased iodine-123 metaiodobenzylguanidine ([123I]-MIBG) imaging. Importantly, the reversal of neuronal remodeling in response to CRT appeared to be beyond that achieved by medical therapy. Also, patients with a less impaired presynaptic adrenergic preservation (or a better sympathetic reserve) showed a better response to CRT.
3.3.3.3 Physical Exercise
Physical exercise has been demonstrated to improve exercise tolerance and quality of life and reduce hospitalizations in heart failure (Flynn et al. 2009; O’Connor et al. 2009). There is also limited evidence that exercise reduces mortality. One of the responsible mechanisms for these beneficial effects is the counteraction of the sympathetic hyperactivity. Heart rate variability, beat to beat variations in time of consecutive heartbeats expressed in a normal sinus rhythm, is frequently used to evaluate the ANS (McMillan 2002). Training has been shown to improve heart rate variability, indicating that the ANS and the sinoatrial node respond dynamically to environmental changes (Larsen et al. 2004; Malfatto et al. 2002; McMillan 2002; Murad et al. 2012; Roveda et al. 2003).
Regular exercise reverses sympathetic hyperactivity in favor of exercise tolerance, through its influence on the different receptors which mediate the sympathico-vagal balance.
Training effects have traditionally been attributed to peripheral rather than central adaptations. A (partial) reversal of structural abnormalities in skeletal muscle such as a decreased oxidative capacity, an impaired leg muscle blood flow with endothelial dysfunction, and a glycolytic fiber type distribution has been demonstrated after physical exercise (Hambrecht et al. 1995, 1997, 1998; Sullivan et al. 1988). This reshift towards an aerobic metabolism may result in a decreased ergoreflex activity with a concomitant reduced activation of the sympathetic outflow (Piepoli et al. 1996; Wang et al. 2010, 2012). Another effect of physical exercise is the restoration of the blunted baroreflex sensitivity (Gademan et al. 2007; Iellamo et al. 2011). The baroreflex is known to buffer the ergoreflex-mediated vasoconstriction (Kim et al. 2005b). This buffering mechanism together with a reduced triggering of the ergoreceptors results in an improved skeletal muscle blood flow which has a positive effect on fatigue complaints.
As ergoreflex activity is also known to influence ventilation, a decrease in its activation through physical exercise has a positive impact on the prognostic important ventilatory inefficiency, typically seen in patients with heart failure (Piepoli et al. 1996). Physical exercise also reduces chemoreceptor activity, another mediator of the ventilatory drive (Li et al. 2008).
Through its impact on the periphery, physical exercise is able to influence the ANS thereby dealing with the two main reasons for exercise intolerance, dyspnea, and fatigue.
3.4 Heart Failure and Reduced Vagal Function
3.4.1 Pathophysiology
Dysfunction of the PSNS has been documented extensively in heart failure in both animal studies and humans. Already in 1971, Eckberg et al. (1971) showed that arterial baroreflex control of heart rate was reduced in patients with left ventricular dysfunction. Moreover, altered vagal control of heart rate appears to be present early in the development of left ventricular dysfunction (Kinugawa and Dibner-Dunlap 1995) and is associated with a poor prognosis in patients post myocardial infarction and in heart failure post myocardial infarction (Kleiger et al. 1987; La Rovere et al. 1998). Despite the recognition of a reduced vagal control in heart failure and its association with worse outcomes, the precise anatomical sites and mechanisms of abnormal vagal control are not very clear (Bibevski and Dunlap 2011). The overall (limited) evidence suggests that the anatomical level of dysfunction seems to lie at the level of the ganglion since postganglionic mechanisms are upregulated and functional (Bibevski and Dunlap 2011). Different non-invasive techniques can be used to measure vagal nerve activity including resting heart rate, heart rate variability, baroreflex sensitivity, and heart rate turbulence (for an extensive review, see Chapleau and Sabharwal 2011).
3.4.2 Effects During Exercise
In normal subjects, vagal control results in a reduction in resting heart rate through its inhibitory effect on the SNS and hyperpolarization of the sinus nodal cells. Via a NO pathway, parasympathetic activation can cause vasorelaxation, but vasoconstriction through its action on vascular smooth muscle has also been described (Olshansky et al. 2008). Apart from the bradycardic effect, a negative inotropic effect resulting in a decreased myocardial contractility has been demonstrated after vagal nerve stimulation (Lewis et al. 2001). As such, parasympathetic activity decreases the cardiac work and myocardial oxygen demand (Buch et al. 2002).
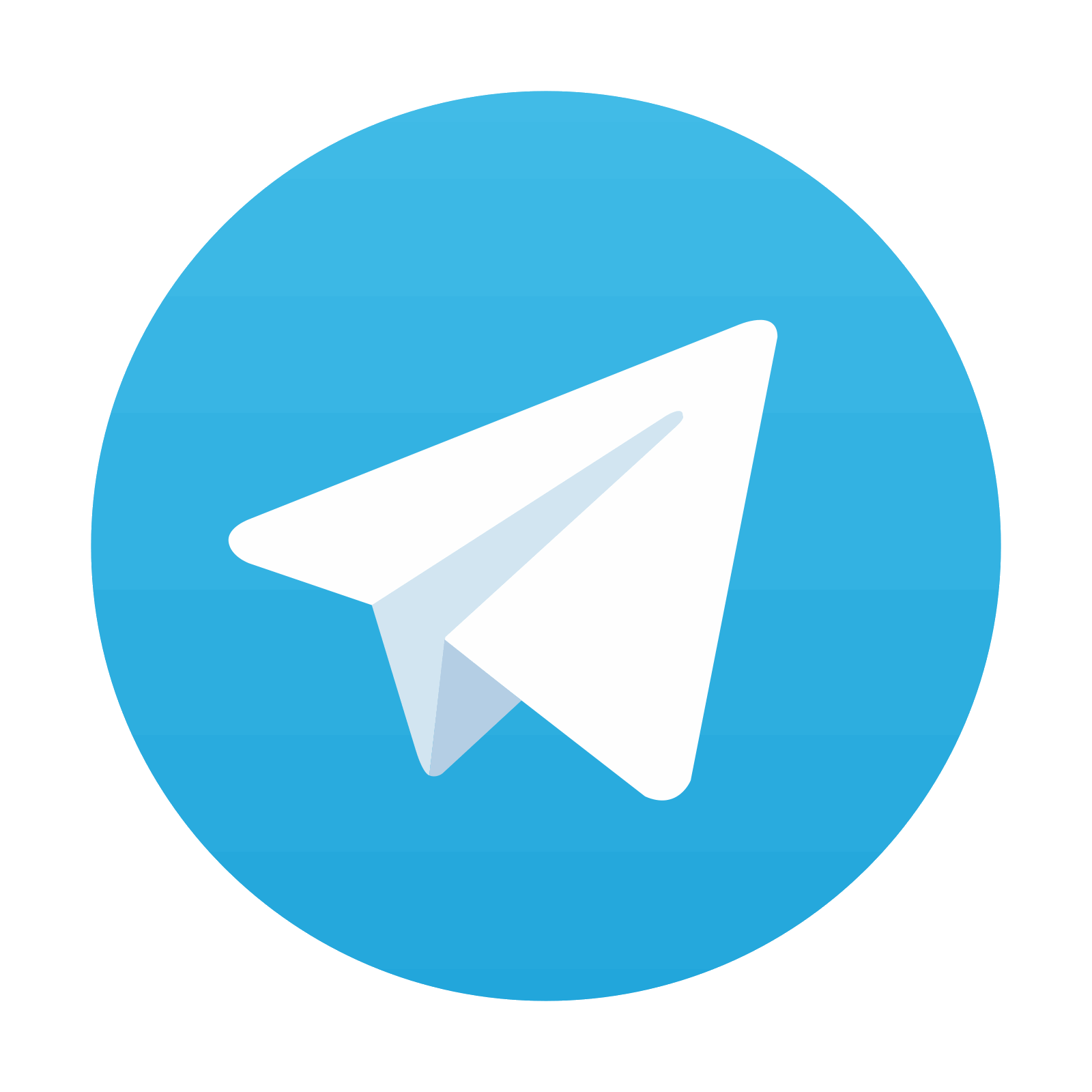
Stay updated, free articles. Join our Telegram channel
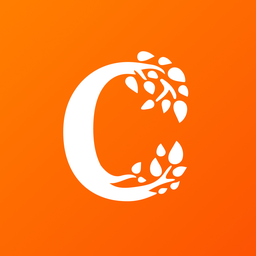
Full access? Get Clinical Tree
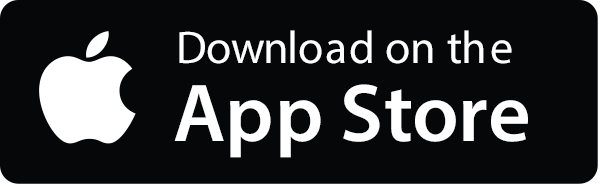
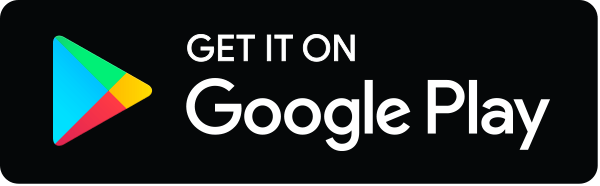