Fig. 11.1
Synthetic polymer scaffold before cell seeding consisting of poly-4-hydroxybutyrate (P4HB) coated polyglycolic acid (PGA) leaflets attached to a Fastacryl® stent. Views from the ventricular side (left) and aortic or pulmonary side (right) are shown. With kind permission from Springer Science + Business Media ([6], p. 1779, 1b, c), Mol A, Driessen NJ, Rutten MC, et al (2005) Tissue engineering of human heart valve leaflets: a novel bioreactor for a strain-based conditioning approach, Ann Biomed Eng 33(12):p 1779, 1b,1c
In order to avoid negative effects from degradation byproducts, a biopolymer like fibrin can be used as a scaffold material. A fibrin gel is a highly hydrated network of entangled protein fibrils in which cells become entrapped, producing a completely biological TEHV [17, 19]. Furthermore, fibrin undergoes controllable, natural enzymatic degradation, which is a significant advantage over synthetic polymer hydrolysis. Fibrin scaffolds are additionally advantageous, because the cell-mediated fibrin gel contraction can be used to achieve fiber alignment and anisotropy similar to that of native heart valve root and leaflets [19]. With this method of TEHV fabrication, cells are suspended in fibrinogen, and the addition of thrombin causes a fibrin gel to form. The suspension can be cast into a mold with the desired geometry, and the cells contract the fibrin gel around the mold surfaces.
While commercial fibrinogen and thrombin have been used in most experiments to date, these components can be isolated from an autologous blood sample. A general process for fibrin-based TEHV fabrication and remodeling is shown in Fig. 11.2. It should be noted that a fibrin gel is much weaker than native heart valve tissue, even after cell-mediated contraction of the fibril network. Therefore, a significant challenge in the production of fibrin-based TEHVs is obtaining sufficient mechanical properties for in vivo function by inducing the cells to convert the aligned fibrin into aligned ECM. For example, Mol and colleagues employed a hybrid approach in which fibrin was used as a cell carrier to seed cells onto a PGA scaffold, exploiting both the stiffness of PGA and the biological properties of the fibrin [6]. However, the high initial stiffness and hydrolytic degradation products may still be problematic in this case.
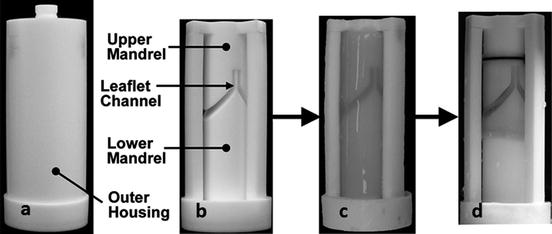
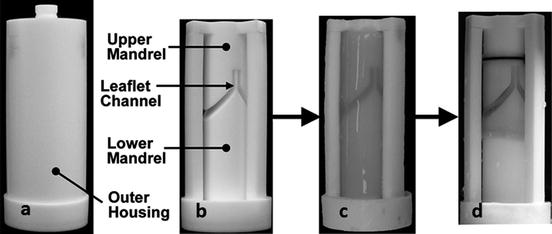
Fig. 11.2
Casting and compaction of a fibrin-based bi-leaflet tissue engineered heart valve (TEHV): (a) TEHV mold with outer housing, (b) mold with outer housing removed, (c) fibrin gel with entrapped fibroblasts cast into TEHV mold, (d) compacted TEHV after 3 weeks of in vitro culture. Adapted from Robinson et al. [19]
In a fairly recent approach, Filho and co-workers developed a method of printing hydrogels in the geometry of a heart valve using a 3D printer. The resultant hydrogel consisted of interpenetrating networks of polyethylene glycol diacrylate (PEGDA) and collagen, and it was photo-crosslinked by UV light upon ejection from the print head [23]. By creating hydrogels consisting of different compositions of a high and low molecular weight PEGDA, they were able to tune the modulus of the printed gels. This enabled them to print a scaffold with spatially varying mechanical properties similar to a native heart valve, although the fabrication process was more intensive than the more common synthetic polymer seeding or biopolymer casting approaches. Subsequently, they used porcine aortic valve interstitial cells that were cultured to populate these collagen/PEGDA scaffolds which were shown to be viable and exhibited spread morphology [24]. As with cell source selection, there are many factors to weigh when selecting a scaffold material, and more detailed discussions of TEHV scaffold materials are provided elsewhere [14, 15, 25].
11.2.3 In Vitro Culture Conditions
The in vitro culture environment can profoundly affect the final TEHV properties. Biochemical molecules such as growth factors, ascorbic acid, and insulin have been shown to have significant effects on the resulting TEHVs. For example, Ramaswamy et al. were able to nearly double the amount of collagen produced per MSC in their synthetic polymer-based constructs by supplementing their standard growth medium with basic fibroblast growth factor and ascorbic acid-2-phosphate [26]. In fibrin-based constructs, Neidert et al. demonstrated that collagen deposition by human dermal fibroblasts could be increased 20-fold by supplementing their medium with transforming growth factor-beta, plasmin, and insulin [27]. Depending on the cell type and scaffold material involved, each laboratory uses different combinations of culture medium and supplements in an attempt to optimize ECM production and maturation.
Traditionally, engineered cardiovascular tissue has been cultured under a normal atmospheric oxygen concentration of 21% partial pressure. However, recent studies of myofibroblasts both in monolayer [28] and 3D culture [29] have shown increased collagen synthesis and crosslinking under hypoxic conditions. In our laboratory, culturing neonatal human dermal fibroblasts (nhDFs) in tubular fibrin constructs in a 2% oxygen environment improved the modulus and tensile strength of the tissue more than threefold compared to controls cultured in normoxic conditions. The combination of a 2% oxygen environment with insulin supplementation was found to improve the ultimate tensile strength and modulus sixfold and fivefold, respectively (unpublished data). Although the idea of hypoxic culture is still relatively new to cardiovascular tissue engineering, it may provide means of improving TEHV properties and reducing the required in vitro culture time.
11.2.4 Bioreactor Conditioning
The development of bioreactors for the mechanical conditioning of TEHVs is a topic of much current research. A TEHV is a complex 3D structure which can be mechanically stimulated in many ways. Flow through a TEHV results in combinations of leaflet flexure and stretch, shear stress, and root distension. Because these structures are so complex, the optimal stimulation protocol is still unknown, yet several research groups have developed different types of bioreactors to improve the mechanical properties of their constructs. For example, recent work has focused on the development of bioreactors which allow the different modes of stimulation to be decoupled and considered individually for simplified systems.
11.2.4.1 Modes of Stimulation
There are several key modes of mechanical stimulation used in conditioning TEHVs; a complex combination of these stimuli exists in vivo in a native heart valve. More specifically, in vivo, mechanical strain is dynamic and is caused by: (1) the pulsatile nature of flow through the heart; and (2) distention of the heart valve root and flexure and stretch of the leaflets resulting in the application of a complicated strain field to the valve. In vitro, mechanical strain can be applied to both the leaflets and the root of the TEHV. The applied mechanical strain can be static or dynamic, and the magnitude and direction of the applied strain must be carefully considered. Another important stimulation mode both in vivo and in vitro is shear stress, which is applied to the root and leaflet surfaces by flow through the valve. The shear stress profile on native heart valves is complicated, spatially varying, pulsatile, and changes with relative cardiac output or function [3]. In simplified engineered tissue geometries the shear stress can be applied in a well-controlled fashion, but when combined with other modes of mechanical stimulation, such as stretch or flexure, the stress profile on the tissue engineered construct becomes time-varying and non-uniform [26].
The flow of blood or culture medium not only provides shear stress, but also aids the transport of nutrients to the cells and may produce interstitial or transmural flow through the root segment of the heart valve. Because TEHV roots can be porous, flow entering the lumen of the valve can flow out both axially and radially, if the bioreactor configuration permits transmural (radial) flow. It has been observed that transmural flow can improve nutrient delivery and waste removal as well as increase the uniformity of nutrients within the tissue. In addition, transmural flow applies shear stress to interstitial cells, whereas axial flow only applies stress directly to cells on the lumenal surface of the TEHV root [30]. Nevertheless, bioreactors for the study of these modes, individually or in combination, must provide well-controlled mechanical stimuli that can be characterized by experiments, mathematical models, or both.
11.2.4.2 Bioreactors for the Study of Mechanical Stimulation Modes
TEHVs can be considered as large tissue constructs requiring significant amounts of space and culture medium, and flow through a TEHV results in a combination of mechanical stimulation modes. Therefore, it is typically not desirable or even possible to use complete TEHVs to study individual mechanical stimuli. For these purposes, researchers to date have used small rectangular pieces of engineered tissue, which can be studied in a controlled and efficient manner. Rubbens and colleagues used a modified Flexercell system to strain myofibroblasts seeded onto a PGA/P4HB scaffold. The system applied vacuum beneath a flexible membrane to which the scaffold was attached, thereby flexing the tissue construct. The applied strain was quantified by placing markers on the flexible membrane and using digital image correlation to map the displacement of the markers during flexion. Using this system they found that uniaxial dynamic strain of 4% at 1 Hz increased collagen crosslinking and glycosaminoglycan (GAG) content compared to statically constrained controls. Interestingly, they did not find collagen production to be upregulated by this dynamic straining protocol [31]. They also explored the effects of strain magnitude, and determined that for these same constructs, 8% dynamic strain had a negative effect on the mechanical properties, thus emphasizing the need to choose straining protocols carefully [32]. With this same system, Rubbens et al. demonstrated that intermittent straining, in which the tissue specimen was subjected to periods of 4% strain at 1 Hz for 3 h alternating with 3 h periods of static culture, increased collagen production, collagen crosslinking, and GAG content, allowing them to achieve improved mechanical properties with a shorter culture time [33].
Engelmayr and co-workers have developed a Flex-Stretch-Flow (FSF) bioreactor to study the effects of cyclic flexure, stretch, and laminar shear flow on rectangular engineered tissue constructs. A schematic of this FSF bioreactor is shown in Fig. 11.3. The constructs of interest include rectangular PGA/PLLA (poly-l-lactide) scaffolds seeded with MSCs which were attached to posts. The distance between the posts was regulated by a linear actuator to flex or stretch the specimens, and flow was driven past the specimens by paddlewheels. With this set-up they were able to independently control flexure, stretch, and flow (three major stimuli on native leaflets) in order to investigate the effects of each mode. A computational fluid dynamics (CFD) model was developed for the system, and the results from the model were compared to the velocity field measured using particle image velocimetry (PIV). Furthermore, they were able to predict the stress and strain fields on the tissue constructs using the results of the CFD model and PIV experiments [25]. Studies with the FSF bioreactor demonstrated that although cyclic flexure and flow applied individually did not significantly improve tissue composition and stiffness after 3 weeks, a combination of cyclic flexure and flow increased the collagen content by 75% over static controls [5]. The extent and nature of these responses to mechanical stimuli are dependent on the culture conditions and cell and scaffold type, so experiments isolating one or more of the stimulation modes on a small segment of relevant engineered tissue provide valuable insight into the optimal conditioning regimen.
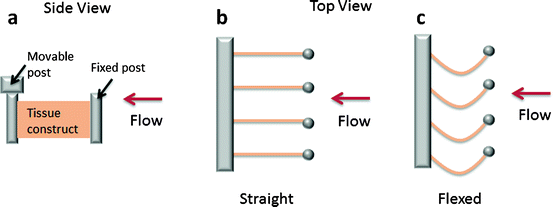
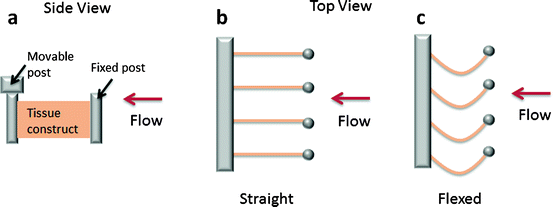
Fig. 11.3
Schematic of flex-stretch-flow bioreactor for conditioning rectangular engineered tissue specimens [34]. Side view (a) and top view (b, c) of tissue constructs mounted in the bioreactor. Specimens were attached to one stationary and one movable post. Displacement of the movable posts by a linear actuator flexed the specimens (c). Cyclical flow of culture medium was driven from right to left by a paddlewheel
11.2.4.3 Bioreactors for TEHVs: Pulse Duplicators
Several groups have designed and implemented pulse duplicator systems to condition entire TEHVs using physiological pulsatile pressure waveforms. The details of such bioreactor systems differ, but all share some key features: (1) a pump to induce pulsatile fluid motion; (2) a medium reservoir to replenish the system; (3) a section in which the TEHV can be mounted; (4) a fluid capacitance for energy dissipation (to mimic arterial elasticity); and (5) a tunable resistance element to control the pressure in the system [35]. In addition, these systems must be sterilizable, allow for gas exchange, fit in a cell culture incubator, and minimize the volume of culture medium used in order to reduce operational cost.
As an example, Hoerstrup et al. developed a pulsatile bioreactor for conditioning their synthetic polymer-based TEHVs that consisted of an air chamber and a medium chamber separated by a silicone membrane. A ventilator was used to pump air into the air chamber, cyclically displacing the silicone membrane and thereby generating pulsatile flow in the medium chamber. The TEHV was mounted onto a tube in the medium chamber, with some culture medium above the valve and some below. By changing the stroke volume and rate of the ventilator, they were able to vary the pressure drop and flow rate across a given valve. With this system they were also able to achieve specified pressures of 10–240 mmHg and flow rates of 50–2,000 mL/min [36]. In one report, TEHVs made from myofibroblasts and ECs seeded on PGA/P4HB scaffolds were subjected to flow conditions gradually increasing from 35 to 50 mmHg and 135 to 750 mL/min over 28 days. Compared to controls that were cultured statically, the bioreactor conditioned TEHVs were more robust, contained more collagen, had higher suture retention strengths, and maintained structural integrity throughout the culture period [10]. An additional benefit of this system was the ability to test the TEHVs at physiological pressures immediately before implantation to ensure function [10]. Similarly, Flanagan and co-workers employed a bioreactor configuration to culture fibrin-based TEHVs with entrapped SMCs and fibroblasts. After 12 days of conditioning at a pressure difference of 20 mm H2O, a flow rate increasing from 160 to 320 mL/min, and pulse frequency increasing from 5 to 10 beats per minute, the leaflets showed increased collagen, fibronectin, and GAG deposition compared to static controls [17].
In another example, Hildebrand et al. developed a pulse duplicator system that was similar to the system described above, but had several novel features including electronic control of all components and a precise pneumatic system for waveform generation. Their system, shown in Fig. 11.4, consisted of a flow loop containing an atrial chamber which filled a ventricular chamber through a mechanical valve. The ventricular chamber was controlled by a pneumatic system which could generate physiological waveforms to eject culture medium through the TEHV mounted downstream. An electronically controlled resistance element was used to achieve the desired system pressure. Pressure and flow through the system were measured using a pressure transducer and ultrasonic flow probe, respectively [37]. In a study to validate the benefits of this system, TEHVs made from MSCs seeded onto PGA/PLLA scaffolds were cultured statically for weeks 1–3 and then subjected to physiological pulmonary valve pressure and flow conditions for weeks 4–6; a fourfold increase in collagen content was observed compared to 6-week static culture samples [26]. It was reported that pulse duplicator systems can have beneficial effects on both TEHV growth and remodeling. However, despite providing well-defined pressure waveforms, the applied mechanical stimuli are complex and difficult to control. It should be noted that the leaflet properties and behavior change as the tissue remodels in vitro, and the mechanical stimuli applied by these systems are time dependent and poorly defined.
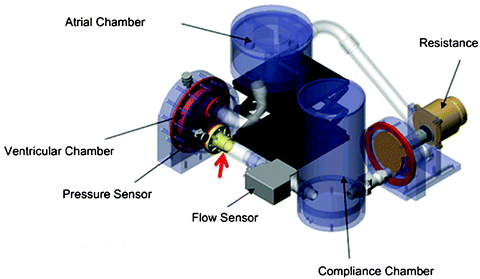
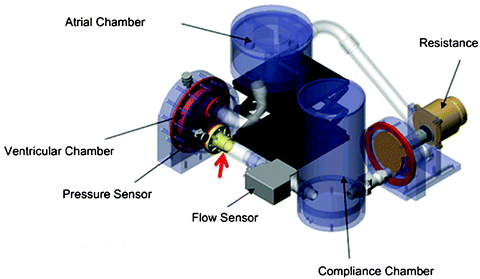
Fig. 11.4
Schematic of the pulse duplicator bioreactor designed by Hildebrand et al. [37]. The location of the tissue engineering heart valve is indicated by the red arrow. Reprinted from [26], with permission from Elsevier, Ramaswamy S et al (2010) The role of organ level conditioning on the promotion of engineered heart valve tissue development in-vitro using mesenchymal stem cells, Biomaterials 31:1114-1125
11.2.4.4 Bioreactors for TEHVs: Controlled Cyclic Stretch
Rather than attempting to replicate physiological operating conditions, several research groups have developed bioreactors to apply well-defined mechanical stimulation to TEHVs. As such, a Diastolic Pulse Duplicator (DPD) bioreactor, a schematic of which is shown in Fig. 11.5, was developed in which cyclic pulses of back pressure were applied to coapting TEHV leaflets by compressing and releasing silicone tubing containing culture medium [6]. The leaflet strain in this system depended on the mechanical properties of the tissue, which varied with time as the tissue matured. In one study, the dynamic strain in the leaflets increased from 8 to 20% from weeks 2 to 4 in culture as the polymer scaffold degraded and the stiffness of the construct decreased [6]. Additionally, no significant improvements in collagen production or mechanical properties were observed in the dynamically strained samples compared to TEHVs which were exposed to constant, low flow rate medium circulation in the DPD system. This lack of improvement may be due to applied strains in the later weeks of the culture period which may have been too large, as the applied load remained constant while the tissue stiffness decreased. Subsequently, Kortsmit et al. proposed a method of implementing a volumetric deformation controlled feedback loop in which the applied load in the DPD bioreactor was automatically adjusted to achieve the desired deformation [38]. By controlling the overall volumetric deformation of the coapting leaflets, they were able to apply defined cyclic strain to their TEHV leaflets.
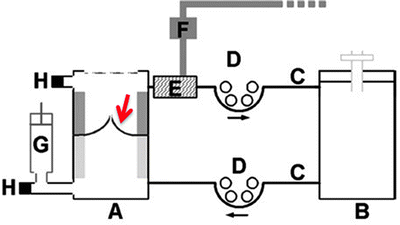
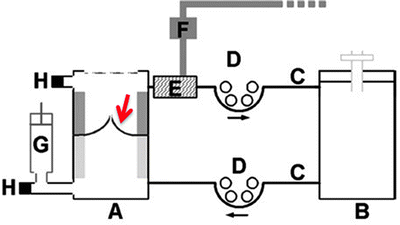
Fig. 11.5
Schematic of diastolic pulse duplicator consisting of a bioreactor chamber (A) and a medium chamber (B). The location of the tissue engineering heart valve (TEHV) is indicated by the red arrow. The bioreactor and medium chambers are connected by tubing (C) and flow is driven by roller pumps (D). Part of the tubing is encased in a polycarbonate cylinder (E), and the injection of air into the cylinder through a magnet valve (F) compresses the tubing and applies cyclic back pressure to the TEHV. A syringe acts as compliance chamber (G), and pressure is monitored by sensors on either side of the TEHV (H). With kind permission from Springer Science + Business Media ([6], p. 1779, 2a), Mol A, Driessen NJ, Rutten MC, et al (2005) Tissue engineering of human heart valve leaflets: a novel bioreactor for a strain-based conditioning approach, Ann Biomed Eng 33(12):p 1779, 2a
Syedain and colleagues designed a controlled cyclic stretch bioreactor for the conditioning of their fibrin-based TEHVs, shown in Fig. 11.6 [7]. Previous work by this group demonstrated improved mechanical properties of vascular grafts subjected to incrementally increasing strain amplitude during cyclic distension [39], and this concept was then adapted for TEHVs. Specifically, a TEHV was placed inside an elastic latex tube and mounted between two end pieces. A reciprocating syringe pump injected medium into both ends of the mounted TEHV simultaneously, causing the TEHV and latex sleeve to distend; then the syringe was refilled as medium was removed from the TEHV. Simultaneously, a peristaltic pump circulated medium in a loop from a reservoir through the TEHV at a constant low flow rate of 10–15 mL/min. The relative strain in the root segment was measured using a laser micrometer, and the circumferential and radial strain in the leaflets was calculated from the displacement of 4 markers located on each leaflet. The distension, and thus the strain in the root and the leaflets, was controlled by the constant stiffness of the latex tubing, as the latex tubing was significantly stiffer than the TEHV at all time points. In this way, strain magnitudes were prescribed and maintained throughout the culture period. Due to the free edge of a leaflet, only its circumferential strain could be prescribed, with a radial strain resulting. Importantly, leaflets of TEHVs exposed to controlled cyclic stretch exhibited improved anisotropy and commissural fiber alignment, similar to those within native valve leaflets. The circumferential tensile strength and modulus of these TEHV leaflets cultured in the bioreactor for 3 weeks were improved over static controls by 97% and 77%, respectively, demonstrating that this system shows promise for improving TEHV properties in a highly controlled manner [7]. It is clear that mechanical conditioning is an important part of in vitro culture, and while an optimal mechanical conditioning protocol has not yet been determined, a wide variety of bioreactors have been developed to condition TEHVs. Therefore, current and future focus on the application of well-defined mechanical stimuli will help researchers optimize mechanical conditioning regimens in order to reproducibly fabricate TEHVs with properties suitable for implantation.
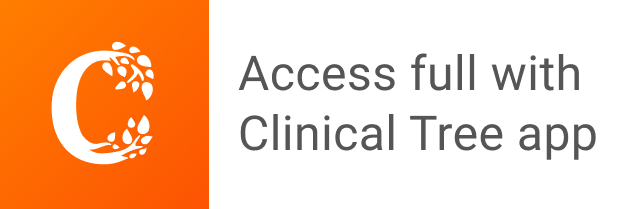