Abstract
The assessment of myocardial performance and hemodynamics in term and premature infants is gaining considerable interest and has led to improvements in our ability to assess the neonatal heart and circulation in health and disease. The use of newer and more novel imaging modalities provides the capability to obtain objective quantitative information that often supersedes the qualitative information provided by conventional methods. Tissue Doppler imaging is a modality that captures information on muscle movement velocity and cardiac cycle event timings using a high temporal resolution. There is an expanding literature in neonates that details the methodology, reference ranges, and diagnostic/predictive capabilities of this modality. A comprehensive understanding of tissue Doppler techniques (that is, what the investigators measure), the confounding factors that influence the measurements (particularly loading conditions), their predictive value, and their limitations can greatly assist in managing both the normal and maladaptive responses in the newborn period. This chapter discusses the novel and emerging methods for assessment of left and right heart function in the neonatal population.
Keywords
myocardial function, neonates, premature infants, tissue doppler imaging
- •
Tissue Doppler imaging (TDI) is a modality that employs the Doppler effect to assess muscle wall characteristics throughout the cardiac cycle including velocity, displacement, deformation, and event timings.
- •
TDI is feasible and reliable in the neonatal population with recent literature describing the normative and maturation values of the various measurements in a wide range of gestational ages.
- •
Those measurements can be used to assess myocardial performance in various disease states, monitor treatment response, and provide important prognostic information.
- •
TDI velocity and deformation assessment measure myocardial performance rather than intrinsic contractility, and as such, the values are highly influenced by loading conditions (in addition to intrinsic contractility). Increased preload increases systolic tissue Doppler velocities while increased afterload reduces those velocities.
The Doppler effect is the term given to the change in frequency of a wave reflected by an acoustic source when there is relative movement between the source and the wave transmitter. It was first described by Christian Doppler, an Austrian physicist, born in Salzburg in 1803. He observed that the frequency of a wave depended on the relative speed of both the source and the observer. This became known as the Doppler effect. The difference between the transmitted and received frequencies can be used to measure the velocity of the moving acoustic source. The use of color flow Doppler in echocardiography was first described by engineers in Washington, although its clinical applicability was soon demonstrated in Japan in 1984 when Doppler waves were used to assess the velocity of blood through the heart.
Traditionally, Doppler-based echocardiography is used in clinical practice to evaluate the velocity of blood flow. The stationary ultrasound probe emits high-frequency waves, which are reflected by moving blood and received by the probe again. The frequency of the emitted waves is different from that reflected back to the receiver within the probe. Each moving cell generates its own Doppler signal, which is scattered in all directions. Signals are reflected back to the ultrasound probe by millions of blood cells. The difference in frequencies is then expressed as both an audible pitch and velocity on the screen. The clinical applications of Doppler include pulse-wave, continuous-wave, and color flow Doppler methods.
More recently, the use of the Doppler effect has expanded to the assessment of heart muscle (tissue) characteristics. This was first demonstrated by Isaaz et al. in their assessment of the left ventricular wall using a pulse wave frequency signal. Tissue Doppler imaging (TDI) captures information using high frame rates (typically greater than 200 frames per second). The high temporal resolution achieved using this technique facilitates the measurements of a wide array of myocardial muscle characteristics, including the velocity of muscle movement during systole and diastole, deformation measurements (also known as strain and strain rate [SR] measurements), in addition to the measurements of the timing of events within the cardiac cycle (systolic and diastolic times/isovolumic contraction and relaxation times). Those measurements can now be derived by pulsed wave tissue Doppler imaging (pwTDI) and color tissue Doppler imaging (cTDI; Fig. 12.1 ).

Unlike conventional methods of functional assessment, which mainly assess changes in cavity dimension and blood flow velocity; namely, shortening fraction, ejection fraction, and blood pool analysis, this modality directly assesses muscle wall characteristics, such as velocity and deformation. In addition, it has shown improved sensitivity over older methods as an intensive care monitoring tool for myocardial dysfunction in critically ill term and preterm infants in conditions such as congenital diaphragmatic hernia and neonatal sepsis. This provides the physician with valuable data on myocardial performance and has the ability to assist in outcome prediction. Unfortunately, like other echocardiography modes, TDI is influenced by loading conditions and, therefore, is not a true surrogate for intrinsic myocardial function (see below).
Principles of Cardiac Function
To understand the relative strengths and weaknesses of all the measurements obtained using TDI, a thorough understanding of the mechanics of ventricular pump performance is required. It is important to distinguish between intrinsic myocardial function (termed contractility) and ventricular pump function (termed myocardial performance). Contractility refers to the crosslinking of the actin and myosin filaments resulting in active myofiber force development and the shortening of sarcomeres. Myocardial performance or pump function describes the overall ventricular pressure development and deformation resulting in the ejection of blood from the ventricular cavity. Myocardial performance is therefore dependent on important physiologic factors: (1) Preload: defined as the amount of blood present in the ventricle at end-diastole before contraction begins. Up to a certain point, higher preload results in greater force generation and improved function (Frank-Starling relationship). Left and right ventricular preloads are dependent primarily on pulmonary blood flow/pulmonary venous return and systemic venous return, respectively. Hydration and diastolic function are the two other important determinants. (2) Afterload: also known as wall stress is defined as the resistance against which the ventricle muscle must contract. This is primarily dependent on vascular resistance, blood viscosity, ventricular muscle wall thickness, and ventricular outflow tract obstructions. Higher afterload results in a reduction in deformation and myocardial performance, particularly in the preterm infant. (3) Contractility: is the intrinsic ability of the myocardial fibers to shorten as described above. This is determined by the efficiency of calcium-dependent crosslinking on the thick and thin filaments within the muscle fiber ( Fig. 12.2 ). The functional measurements obtained using TDI predominantly assess myocardial performance rather than intrinsic function, and as such, interpretation of values obtained during assessment should be done in the context of the clinical situation and loading conditions.

Pulsed Wave Tissue Doppler Velocity Measurements
Tissue Doppler (TD) velocities can be acquired by spectral analysis using a pulsed wave Doppler technique. The muscle tissue wall moves at a significantly slower velocity and a higher decibel amplitude range than blood, thus facilitating a high temporal resolution with minimal artifact from blood. Recent advances have enabled the distinction between the faster moving blood (>50 cm/s) and the slower moving muscle tissue (<25 cm/s). PwTDI assesses longitudinal velocity of a ventricular wall segment from base to apex, providing a measure of systolic function that is recorded as the peak systolic velocity of the myocardial muscle (s` wave). The systolic wave is usually preceded by a short upstroke during isovolumic contraction. In addition, a measure of diastolic performance can be obtained as the ventricular wall moves away from the apex in the opposite direction. The diastolic wave is biphasic and is recorded as the peak early diastolic velocity (e` wave) and the late diastolic peak velocity (a` wave), which reflects the active ventricular relaxation and atrial contraction phases of diastole, respectively. The diastolic waves are usually preceded by another short upstroke during isovolumic relaxation time. The duration of the isovolumic relation and contraction phases, in addition to the systolic and diastolic times, also can be accurately obtained using this modality (see Fig. 12.1 ).
Color Tissue Doppler Velocity
cTDI uses phase shift analysis to capture atrioventricular annular excursions. Compared with pwTDI, cTDI provides the ability to visualize multiple segments of the heart from one single view. It measures mean rather than peak systolic and diastolic velocities. As a result, velocities obtained using this technique are generally 20% lower in systole and diastole compared to pwTDI. Therefore, the two methods are not interchangeable. cTDI does have the advantage of combining the high temporal resolution seen with pwTDI, with a high spatial resolution. In addition to this, myocardial velocities recorded at the left and right ventricular bases, and septal wall, can be obtained from a single image for offline analysis. A comparison between left and right ventricular function can therefore be performed. Muscle tissue at the base moves at a higher velocity than that closer to the apex. cTDI can be used to assess this velocity gradient across the wall of interest ( Fig. 12.3 ). As with other TDI modalities, images are obtained from the apical four-chamber view. Eriksen et al. compared cTDI with tricuspid and mitral annular plane systolic excursions in a cohort of preterm infants. They found that the values obtained from cTDI were lower and more dependent on image quality than excursions by gray-scale M-mode. Another potential disadvantage to cTDI is poor reproducibility as reported in some studies. Data on cTDI values and clinical applicability in the neonatal setting are limited. This is likely due to the need for offline analysis to obtain those values when compared with pwTDI, where data can be acquired at the bedside. The data presented below relate only to pwTDI.

Measurement of Tissue Doppler Imaging Velocities
Accurate TDI velocity measurements are highly dependent on obtaining good quality images. This may be challenging in the neonatal setting, particularly in premature infants where lung artifact can interfere with obtaining clear images of the walls of interest. Images are usually obtained from an apical four-chamber view. The sector width of the field of view is usually narrowed to only include the wall of interest. This ensures that the temporal resolution is enhanced and a frame rate of over 200 frames per second is obtained. A pulsed wave Doppler sample is placed at the base of the left ventricular free wall, the base of the septum, and at the base of the right ventricular free wall. The sample gate is narrowed to only capture the velocity of the area of interest (usually 1 to 2 mm). It is crucial to maintain an angle of insonation of less than 20 degrees to prevent underestimation of velocities. TDI velocity measurement modality will only assess muscle movement parallel to the probe beam ( Fig. 12.4 ). This is a limitation of this modality as muscle movement perpendicular to the line of interrogation will not be assessed and as a result, TDI velocity measurements are reserved for longitudinal (base to apex) muscle tissue movement.

As outlined earlier, TDI velocity assessment measures myocardial performance rather than intrinsic contractility, and as such, the values are highly influenced by loading conditions (in addition to intrinsic contractility). Increased preload increases systolic TD velocities, while increased afterload reduces those velocities. Therefore interpretation of those measurements must take into account made the loading conditions likely to be present in the clinical situation. This has important implications for therapeutic interventions where, in some instances, it may be more beneficial to improve preload (using volume support) or reduce afterload (using lusitropic medication) rather than targeting an improvement in intrinsic contractility ( Fig. 12.5 ). In addition, it is important to recognize that TD velocity imaging cannot distinguish between active muscle movement and translational wall motion (a nondeforming segment tethered to a functioning segment). TD velocities may be falsely elevated as they interrogate motion at a single point in the muscle wall with reference to the ultrasound transducer. Therefore this movement can be influenced by translational motion. Deformation imaging (see later) can easily differentiate between the two.

Clinical Application of Tissue Doppler Imaging Velocity Measurements
The use of TDI in the neonatal setting has significantly expanded in recent years. TDI assessment is very feasible in small infants and has been validated in term and premature neonatal populations as well as in fetuses. Reference values for both term and preterm infants are shown in Table 12.1 . Several studies have documented serial changes in those functional measurements over the first day of life and up to 36 weeks’ postmenstrual age. TD velocity imaging is dependent on the gestational age, with lower gestations exhibiting lower myocardial velocities in both systole and diastole. Reduced myocardial velocity is also present during the transitional period from the fetal to the neonatal circulation and increases over the first few postnatal weeks. This is likely due to the changing loading conditions seen during this period, which become more favorable. In premature infants, TDI velocities have been used to predict clinical deterioration following patent ductus arteriosus (PDA) ligation and as a guide to institute targeted therapy. In addition, they can be used to assess treatment response in this scenario when conventional measures of function, including shortening and ejection fraction, are not sensitive enough. Incorporating TDI for the assessment of myocardial performance in the setting of a PDA during the first few days of age may facilitate a more targeted approach to PDA treatment and facilitate the development of prediction markers for adverse outcomes. Of note is that there is also an emerging association between lower diastolic function measured using TDI and chronic lung disease in premature infants.
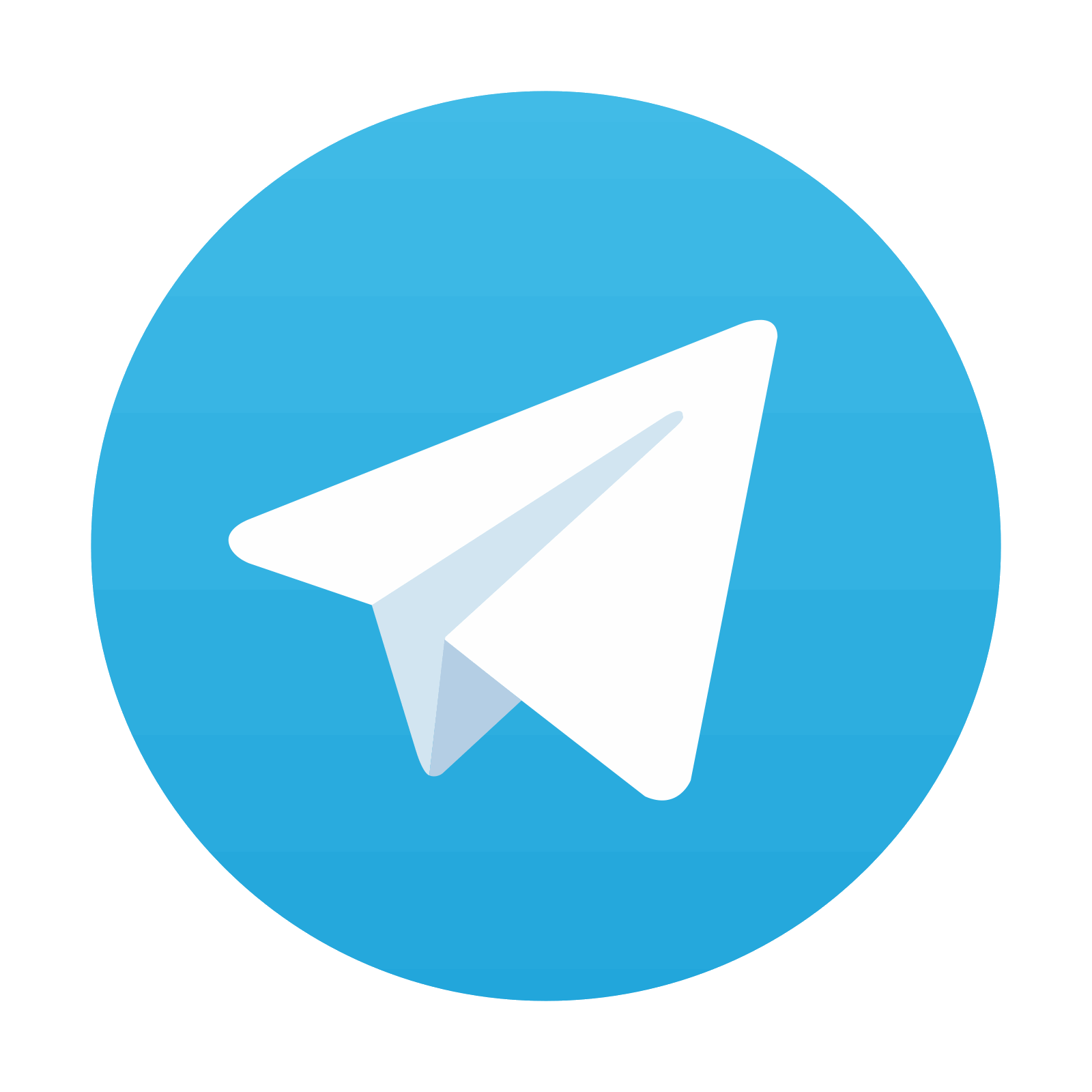
Stay updated, free articles. Join our Telegram channel
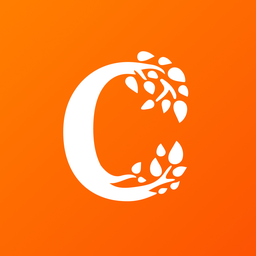
Full access? Get Clinical Tree
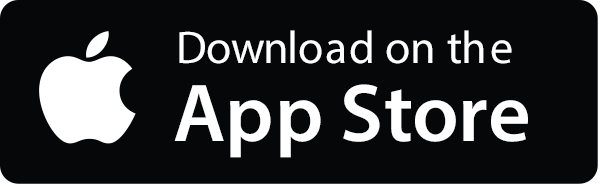
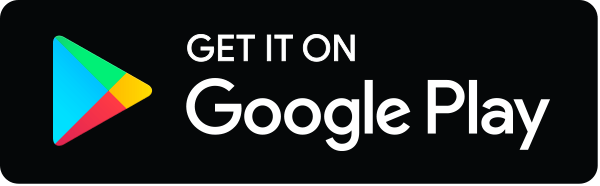
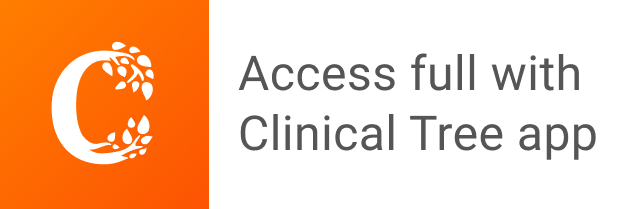