Introduction
Imaging plays a major role in the detection, diagnosis, and serial evaluation of thoracic disease. The appropriate use of imaging techniques requires some basic understanding of the technical aspects of and abnormal findings visible with different imaging techniques and of the diagnostic accuracy of these techniques. It is not the intent of this chapter to provide a complete description of the techniques involved or an encyclopedic catalogue of radiographic abnormalities of chest diseases. Rather, this chapter is intended to provide a general survey of the imaging methods available, their most common indications, and certain principles concerning their use.
Since the late 1990s, there have been remarkable advancements in the effectiveness of cross-sectional imaging techniques for the diagnosis of thoracic diseases, particularly with the development of multislice computed tomography (CT), as well as improvements in magnetic resonance imaging (MRI) and ultrasonography. In many instances, cross-sectional methods have supplanted radiography for the diagnosis of chest diseases. As with any imaging method, the decision to utilize cross-sectional imaging should be based on consideration of the patient’s clinical problem and the results of other imaging and laboratory testing.
Chest radiography still plays a fundamental role in the diagnosis of thoracic disease. Chest radiography is usually the initial imaging procedure performed when chest disease is suspected, and despite the proliferation of other imaging methods, chest radiography remains one of the most frequently performed radiographic examinations in the United States.
The thorax is difficult to image with radiographic techniques because of large regional differences in tissue density and thickness. For example, with standard radiography, the number of x-ray photons passing through the lungs is more than 100 times greater than the number of x-ray photons penetrating the mediastinum. The dynamic contrast range of conventional film-screen radiography is insufficient to demonstrate this range of x-ray photon transmission properly; with standard radiographic techniques, the use of exposure high enough to display the mediastinum and the subdiaphragmatic regions usually results in overexposure of the lungs ( Fig. 18-1A ). Conversely, an exposure designed to provide the best visualization of the pulmonary parenchyma (see Fig. 18-1B ) is normally too light to visualize mediastinal anatomy. One of the advantages of digital imaging is that displayed contrast and brightness are largely independent of the kilovoltage and milliamperage values used to obtain the examination; both contrast and brightness can be manually adjusted by the user after the image has been obtained. Furthermore, the dynamic contrast range (latitude) of digital radiographic techniques exceeds that of film-screen methods by a factor of 10 or more.

Chest radiography has been in use since the discovery of x-rays, and evolutionary developments in radiographic technology have addressed some of the fundamental limitations of radiographic techniques. Whereas film-screen projection radiography was the primary method by which chest radiography was performed for many years, digital radiography has largely supplanted film-screen techniques, providing advantages such as image postprocessing and electronic storage of the radiographic data. The latter is particularly important because it allows for simultaneous access to imaging studies by multiple providers and transmission of the data over long distances quickly.
Meticulous attention to technique is essential. Regardless of the method of recording the image, whether through standard film-screen radiography, image intensification, or digital recording, poor quality control leads to degradation of diagnostic information. Unfortunately, many technically inadequate radiographs are produced, leading either to repeat studies with additional patient radiation exposure or to interpretation of the poor images, increasing the chance of diagnostic errors.
Indications for the use of chest radiography are protean and include the assessment of both acute (e.g., pneumonia) and chronic (e.g., chronic obstructive pulmonary disease [COPD]) lung diseases, assessment of dyspnea or other respiratory symptoms, evaluation of treatment success for patients with acute lung disease, follow-up of patients with a known chronic lung disease, monitoring of patients in intensive care units (ICUs), diagnosis of pleural effusion, screening for asymptomatic diseases in patients at risk, monitoring patients with industrial exposure, preoperative evaluation of surgical patients, and as the initial imaging study in patients with known or suspected lung cancer and other tumors, vascular abnormalities, and hemoptysis. Abnormal radiographic findings can be quite subtle, however, and, in many circumstances the sensitivity and specificity of chest radiography is limited. In such situations, other imaging studies, especially chest CT, are performed to investigate abnormalities visible on radiographs or to evaluate patients considered high risk for a particular condition, but with normal chest radiographic results.
The utility of chest radiography has been studied in a number of clinical settings, and the appropriate criteria for their use have been determined by the American College of Radiology (ACR, http://www.acr.org/Quality-Safety/Appropriateness-Criteria/Diagnostic/Thoracic-Imaging ). Although a detailed analysis of the diagnostic accuracy of chest radiographs in all instances is beyond the scope of this chapter, for illustrative purposes their utility and limitations in several specific clinical settings are reviewed.
Chest Radiography: Techniques
The goal of chest radiography is to maximize diagnostic information through optimal film quality, while limiting radiation exposure to the patient. Although there are minor disagreements among experts, virtually all definitions of image quality include three general factors: radiographic contrast, resolution, and image noise. These are interrelated, and the ultimate quality of the radiograph is determined by the worst of the factors involved in its production.
Major Factors Affecting Image Quality in Standard Film-Screen Radiography and Digital Radiography
Standard analog film-screen radiography has been employed for the production of chest radiographic images for many years, but has largely been supplanted by digital techniques. With film-screen radiography, an x-ray photon is absorbed in a screen containing a photostimulable coating, resulting in the production of multiple light photons that, in turn, expose the actual film. This method differs from that used by digital radiographic techniques for image creation; the differences between film-screen and digital radiographic techniques are reviewed below. Nevertheless, the following discussion of technical factors impacting imaging quality applies to both methods.
Radiographic Contrast
Radiographic contrast, or contrast resolution, may be defined as the magnitude of signal difference between the imaged object and its surroundings in the displayed image, and it is principally determined by components of the system, chiefly the energy of the x-rays, and the degree to which secondary radiation (scatter) is eliminated. A film-screen combination possessing an intermediate or wide gray scale (latitude) is preferable to one producing extreme contrast ; such “wide latitude” is intrinsic to digital radiographic production methods and, as will be discussed shortly, is one of the advantages digital imaging enjoys over older analog fim-screen techniques. Although very “white-and-black” radiographs appear to demonstrate excellent detail, differentiation of structures differing slightly in density, such as the pulmonary vessels and the background lung, is better achieved with a wide-latitude film-screen combination or digital radiography. Wide-latitude film-screen combinations or digital radiography also better display the wide range of densities present in the thorax.
When an object is radiographed, some photons are absorbed or scattered, whereas others pass unaffected through the object ( eFig. 18-1A ). If the object is close to the detector, exposure from scattered radiation degrades image contrast and detail. Because production of scatter radiation varies with the volume of tissue being imaged, the use of devices, referred to as “collimators,” to restrict the beam size to the area being studied reduces scatter radiation. The further addition of filtration to the x-ray tube in the area of the collimator prevents low-kilovoltage radiation from reaching the patient, thus reducing the radiation absorbed by the patient without compromising diagnostic information.
Scattered radiation can also be removed by placing an antiscatter grid between the patient and the x-ray cassette with film-screen systems ( eFigs. 18-1B and 18-2A ). Such grids permit the passage of the parallel primary x-ray photons to expose the detector while absorbing radiation scattered at an angle to the primary beam; the grid also absorbs some primary photons, making it necessary to increase the exposure technique to maintain the correct film density. Similarly, an exposure increase is also required when using grids with digital detectors to compensate for attenuation of the primary radiation beam by the grid. Care must be taken to ensure that the grid is of the proper type and is properly aligned to the x-ray beam. Placement off center, angulation, or the use of a grid focused at the wrong distance may affect the symmetry of radiograph exposure or even render it uninterpretable. Scatter radiation may also be reduced by increasing the distance between the detector and the patient, a process referred to as “creating an air gap” ( eFigs. 18-1C and 18-2B ). Some digital imaging systems, such as scanned-slot digital radiography detectors, have intrinsic scatter rejection capability and therefore do not require the use of a grid.
Spatial Resolution
Spatial resolution reflects the ability of an imaging system to allow two adjacent structures to be visualized as distinctly separate. Spatial resolution of film-screen combinations in common use is 10 to 12 line pairs/mm and is primarily determined by the thickness of the screen employed (thicker screens are associated with poorer spatial resolution). Other technical issues are responsible for limiting spatial resolution in digital radiography systems. A number of other factors may contribute to the reduction of the final resolution achieved on a chest radiograph produced by either film-screen or digital techniques. The use of large focal spots, particularly in thick body parts such as the thorax, degrades resolution by producing “edge unsharpness” or blur due to penumbra effects. Conversely, the limited capacity of a very small focal spot dictates a long x-ray exposure, and body motion can degrade spatial resolution, also causing edge unsharpness. A compromise choice in x-ray focal spot size of 1 mm or less is recommended. Lengthening of exposure duration causes degradation of spatial resolution because of insufficient power of the x-ray generator or deficiency of the heat capacity of the tube. Ideally, exposure duration should not exceed 25 msec and, for frontal projections, should be considerably less. A tube-detector distance of at least 6 feet (1.8 m) is usually used to reduce magnification and image blurring (see eFig. 18-2 ).
Noise
All imaging systems are limited by noise, which, in radiography, may be defined as fluctuations within the image that do not correspond to variations in x-ray attenuation in the imaged object. In radiography, noise is frequently referred to as “quantum mottle.” Noise is primarily determined by the number of x-ray photons used to produce the image, although factors intrinsic to the imaging systems contribute to image noise. The fewer the number of photons, the noisier the image will be. With a given film-screen or digital system, attempts to increase speed generally result in decreased resolution because of mottle.
X-ray screens that employ rare earth phosphors and have the property of converting x-ray photons to light more efficiently have been developed. When used with properly matched films, these rare earth screens double or triple the speed at which a properly exposed radiograph can be made. They do not increase the noise of the system significantly and they permit reduction of radiation exposure to the patient by one half or more.
American College of Radiology Standards
The ACR standards for performing adult chest radiography ( http://www.acr.org/~/media/ACR/Documents/PGTS/guidelines/Chest_Radiography ) specify the use of at least a 72-inch tube-film distance, a tube focal spot not to exceed 2 mm (0.6 to 1.2 mm recommended), rectangular collimation and beam filtration, a high-kilovoltage technique (120 to 150 kVp) appropriate to the characteristics of the film-screen combination, a film-screen speed of at least 200, an antiscatter technique (grid or air gap) equivalent to a 10 : 1 grid (preferably 12 : 1), and a maximum exposure time of 40 msec. The ACR also specifies a maximum mean skin entrance radiation dose (0.3 mGy/exposure). Guidelines for the production of digitial chest radiographs have been published.
Portable Radiography
The proliferation of ICUs and the increased use of patient monitoring for life-support devices have significantly increased the number of portable radiographs obtained. As indicated earlier, these examinations are necessary to determine whether catheters, endotracheal tubes, intra-aortic balloons, and various other devices have been correctly placed ( eFigs. 18-3 to 18-7 ). Portable radiographs are also essential for assessing responses to therapy and for surveying for the presence of new thoracic disease.
Even under ideal circumstances, the quality of the radiographs obtained with portable techniques does not approach the standard of those made in the radiology department ( eFig. 18-8 ). Portable examinations are generally made at a focal spot–detector distance of less than 72 inches, resulting in penumbral blurring, edge unsharpness, and degradation of fine detail. The routine use of the anteroposterior (AP) projection, with the recording device adjacent to the patient’s back, magnifies the cardiac silhouette and other anterior structures. When possible, the radiograph should be obtained with the patient in the sitting position, but this is frequently not feasible. Images obtained with the patient recumbent compromise the detection of pleural effusions and pneumothorax, as well as the assessment of the gravitational distribution of pulmonary blood flow based on vessel size and lung zone opacity.
None of the several different types of mobile generators available is ideal. Exposure duration is difficult to control and is relatively long, and motion blurring degrades the image. Because of these limitations and the frequent need for immediate viewing of chest radiographs, storage phosphor-computed digital units are widely used for ICU radiography (see later discussion). The storage plate phosphor’s ability to provide relatively even density for overexposed and underexposed films and its ability to adjust the images digitally to visualize mediastinal or pulmonary parenchymal areas are well suited for ICU portable radiography.
The ACR standards for performance of portable chest radiographs ( http://www.acr.org/~/media/ACR/Documents/PGTS/guidelines/Portable_Chest.pdf ) are relatively forgiving. A tube-film distance of 40 to 72 inches is recommended, closer to the latter preferred. From 70 to 100 kVp is recommended if a grid is not used, whereas more than 100 kVp may be used in conjunction with a grid. Exposure times should be as short as feasible to reduce motion artifacts.
Digital (Computed) Radiography
The development of reusable radiation detectors along with advances in electronics and computer technology enabled the development of a new type of radiographic imaging: digital radiography and computed radiography. These systems are fundamentally different from film-screen radiography in that image detection can be completely separated from the method of image display and, because the images are digital in format, they can be subjected to significant postprocessing to improve diagnostic information. Also, digital image receptors have a much wider range of sensitivity, or latitude, compared with standard film-screen combinations (10,000 : 1 rather than 100 : 1), and their response to radiation is linear over this entire range. Within these large limits, image contrast is independent of exposure. Furthermore, digital images can be displayed, transmitted, and stored electronically, and digital systems allow for faster patient throughput, increased dose efficiency, and possibly reduced x-ray exposure compared with film-screen techniques. These advantages have allowed digital radiography to supplant film-screen radiography in many radiology facilities.
Storage Phosphor Systems
A commonly employed system of digital radiographic imaging, known as computed radiography (CR), uses imaging plates that are exposed by conventional radiographic equipment and can be used like any x-ray cassette. The reusable plates are coated with a photostimulatable phosphor that absorbs energy and retains a latent image when exposed to x-rays. The stored energy of the latent image is released as light when the plate is scanned by a laser in an image plate reader. The light produced is measured and digitized, yielding an image that can be subjected to contrast and spatial frequency enhancement and can be stored on magnetic or optical disks, viewed on monitors, or printed on film with a laser printer ( eFig 18-9 ). The imaging plates are erased by exposure to light and may be reused almost immediately.
The resolution of digital images depends on the matrix size used. Spatial resolution is less than that of film-screen combinations (2.5 to 5 line pairs/mm), depending on the size and type of plate used to capture the image. The unit can be set to perform automatic processing by preprogramming parameters for certain examinations or may be postprocessed manually by the radiologist to enhance various specific image features. The contrast scale can be altered, and the optical density of the entire image, or of various areas, can be changed. Edge enhancement can be obtained by amplifying high frequencies to visualize minute pulmonary detail. For the thorax, the automatic processing can be preset to produce a pair of images: one resembling a conventional film-screen radiograph and the other exhibiting varying amounts of edge enhancement ( eFig. 18-10 ).
An obvious advantage is that the images can be immediately transmitted electronically and viewed at a workstation in the emergency department, ICU, operating room, or other area where online viewing is required. Because the images are stored electronically, they may be recalled for comparison with subsequent studies, and they may be printed for conventional display at any time. High-resolution display units (2000-line) allow displayed images with excellent spatial resolution.
Clinical Efficacy
A large number of studies have compared digital imaging systems with conventional film-screen radiographs in both measured physical performance and interpretive accuracy. It is generally agreed that the denser portions of the thorax and the lung in front of or behind these dense areas are seen significantly better with digital systems. There is disagreement concerning visualization of fine lung detail, line shadows, pneumothoraces, and interstitial lung disease. An important consideration is that digital recording systems have a very wide exposure latitude, and adequate images can be obtained with a wide range of exposures, which reduces the need for repeat imaging.
Factors other than image quality have played a preeminent role in the dissemination of digital techniques. Among these are immediate availability of the images, ease of storage and retrieval, interaction with hospital electronic information systems, and cost of film and file room operation. CT, MRI, ultrasonography, and nuclear medicine studies already are digital. The completely digital imaging department is now common and will likely be ubiquitous in the near future. Digital imaging also enables the use of computer-aided detection teachniques, particularly in the setting of mammography, chest radiography, and chest CT.
Radiographic Views and Techniques
Routine Examination
A routine chest radiographic examination in an ambulatory patient usually consists of posteroanterior (PA) and left lateral projections. The utility of routine lateral radiographs, however, has been questioned. After analyzing more than 10,000 radiographic chest examinations obtained routinely in a hospital-based population, Sagel and associates concluded that the lateral radiograph could be safely eliminated in the routine examination of patients 20 to 39 years of age. Conversely, they and others have concluded that the lateral radiograph should be obtained in patients with suspected chest disease and in screening examinations of patients 40 years of age or older ( Fig. 18-2 ).

Expiratory Views
Conventional radiographs are made at total lung capacity (i.e., full inspiration), thereby permitting the greatest volume of lung to be evaluated for possible pathology and providing the most contrast between intrapulmonary air and normal and abnormal intrathoracic structures ( Fig. 18-3 ). However, localized or generalized air trapping in the lung or pleural space is more easily detected (and sometimes only detected) on a radiograph made during expiration. The normal lung diminishes in volume and increases in density with expiration. Areas of trapping usually retain their lucency and volume regardless of the phase of respiration. With unilateral or localized air trapping, mediastinal shift ( Fig. 18-4 ) and failure of normal elevation of the hemidiaphragm on the affected side are frequently apparent only on expiration. Small pneumothoraces, difficult to visualize and frequently overlooked on inspiratory radiographs, appear larger and more apparent on the expiratory study. As the thorax and underlying lung diminish in volume, the lung becomes denser, whereas the pneumothorax remains essentially unchanged in size, thus occupying a greater proportion of the deflated hemithorax, thereby being outlined more clearly by denser pulmonary parenchyma ( Fig. 18-5 ).



Other causes of localized or unilateral lucency on the radiograph are differentiated from air trapping by the expiratory study. Among these are technical causes such as patient rotation ( eFig. 18-11 ), miscentered x-ray beam or grid, and “the anode-heel effect,” an artifact of asymmetrical generation by the anode of the x-ray tube. Chest wall abnormalities, either congenital or postsurgical ( Fig. 18-6 ), can produce unilateral lucency. Undetected areas of atelectasis with compensatory overexpansion of portions of the lung and primary vascular disease, such as pulmonary embolus (see eFigs. 57-7 and 57-9 ), may also produce lucency. None of these causes of pulmonary lucency will show trapped air on the expiratory radiograph.

Decubitus Views
Decubitus radiography is performed by placing the patient in the recumbent position, usually lying on one side and then the other. The x-ray exposure is made with a horizontal beam in either the AP or PA projection.
The technique is useful for determining the presence or absence of free fluid in the pleural space or parenchymal cavities, for estimating the size of effusions, and for diagnosing pneumothorax in patients who are unable to sit or stand. Free fluid gravitates to the dependent portion of the thorax, which in the decubitus patient lies against the lateral rib cage of the dependent hemithorax ( Fig. 18-7 ) or the mediastinum of the contralateral side; pneumothorax behaves in the opposite fashion. In the typical AP supine chest radiograph, free fluid will layer posteriorly and manifest as an increase in density involving the entire hemithorax. Air within the pleural space will collect anteriorly and is often difficult to detect. The proper use of decubitus radiography will demonstrate the presence of free fluid, pneumothorax, or both ( Figs. 18-8 and 18-9 ). When a portable study must be performed with the patient in bed, it may be technically difficult to obtain high-quality radiographs of the dependent portion of the hemithorax due to the underlying bed, clothes, or other inconveniences. For this reason, bilateral decubitus studies may be obtained. Even in patients in whom PA and lateral erect radiographs can be performed, subpulmonic effusions may be difficult to detect, and no fluid meniscus may be visualized. Unless the fluid is completely loculated, a decubitus study will demonstrate its presence and size (see Fig. 18-9 ). As little as 20 mL of fluid can be seen in the decubitus position.



Lordotic Views
Radiographs made in the lordotic position can be of value in demonstrating lesions in the immediate subclavicular region or partially hidden by the clavicle ( eFig. 18-12 ). This is particularly true if these lesions are located posteriorly. In the lordotic view, which is most frequently obtained in the AP projection, the clavicle, being an anterior structure, is projected above the lung apex, and the subclavicular lung regions are well visualized (see eFig. 18-12 ). The lordotic view may also be used to confirm middle lobe or lingular atelectasis. A lordotic view centered over the lower portion of the chest will project the atelectatic lobe or segment so that it directly faces the x-ray beam, and it will be easily visualized as a dense triangular shadow.
Oblique Views
Occasionally, shallow oblique views are valuable in sorting out superimposed shadows and in visualizing pulmonary parenchymal opacities obscured by superimposition from the heart, mediastinum ( eFig. 18-13 ), or portions of the bony thorax. In general, it is more rewarding to study these opacities with CT.
Fluoroscopy
Fluoroscopy at one time was commonly employed either as the primary method of radiologic examination of the chest or as an adjunct study to standard radiographs. Its use over the past several decades has diminished considerably. However, there remain a few situations in which fluoroscopy can provide information that is difficult to obtain by other means, such as for the detection of abnormalities of diaphragmatic motion that result from conditions affecting the phrenic nerve ( ). The major use of chest fluoroscopy in current medical practice is as a guide for interventional procedures such as catheter angiography and needle or transbronchial biopsies.
Bronchography
Contrast bronchography, formerly a fairly common thoracic examination, has been replaced by fiberoptic bronchoscopy and either CT or high-resolution computed tomography (HRCT) of the lung. In patients with suspected bronchiectasis, HRCT and CT are as sensitive and are safer, more easily obtained, and much more pleasant to undergo than bronchography.
Pulmonary Angiography
Pulmonary angiography has traditionally been employed primarily for the detection or exclusion of pulmonary embolism ( eFig. 18-14 ; see Fig. 57-7 , Fig. 57-11 , Fig. 57-16 , ). Although it has been traditionally regarded as the “gold standard” for making the diagnosis of pulmonary embolism, it has limitations and tends to be underutilized. Other situations in which pulmonary angiography has been employed include the diagnosis and embolization of pulmonary arteriovenous malformations ( eFig. 18-15 ; see Fig. 19-14 ; and Chapter 61 ), pulmonary arterial foreign body retrieval, pulmonary vasculitis ( eFig. 18-16 ; see Chapter 60 ), and occasionally, the delineation of the anatomy of pulmonary vessels before lung surgery. The study is performed by rapidly injecting intravenous contrast material through a catheter and imaging the lung while the contrast material traverses the arteries and veins. Most frequently, the catheter is inserted into the femoral vein percutaneously and then guided into the pulmonary artery under fluoroscopic control.
Angiograms may be done with the catheter in a main branch of the pulmonary artery or more selectively in distal vessels. The radiographic contrast material is injected at the rate of approximately 10 to 25 mL/sec, depending on the vessel size. The volume of contrast depends on the location of the catheter. Between 40 and 60 mL of contrast material is usually injected into the right or left main pulmonary artery; half this amount of contrast is needed with digital subtraction techniques. Selective injections made within the more distal pulmonary arteries require smaller volumes. If the angiogram is performed for the detection or exclusion of pulmonary embolism after an abnormal but nondiagnostic perfusion scan, the location of the perfusion defect on the scan may act as a “road map” for the angiographer, and selective or superselective injections proximal to the area of perfusion deficit may be performed.
Unless the angiogram is done with a balloon occlusion technique, serial recording is necessary. In the past, rapid film changers were used with an exposure rate of 3 or 4/sec, but such “cut-film” techniques have been replaced by digital subtraction angiography. Filming is usually carried out for several seconds after injection to permit visualization of the pulmonary veins, the left side of the heart, and the thoracic aorta.
It is frequently necessary to image in several different projections to confirm or exclude pulmonary embolism. Oblique or lateral projections may be desirable. If the angiographic suite contains biplane equipment, the number of necessary contrast material injections can be diminished by imaging in two orthogonal planes after a single injection of contrast medium. Magnification angiography may show emboli in vessels that are too small to be seen clearly on standard studies.
For a definite diagnosis of pulmonary embolism, it is necessary to visualize the embolus as either a filling defect (see eFig. 18-14 ) within a vessel or the trailing edge of a thrombus. Other abnormalities should not be regarded as diagnostic of embolism.
Complications and death are rare, fortunately. Significant complications are seen in less than 5% of patients, and 0.1% to 0.5% of patients die undergoing angiographic studies to evaluate suspected pulmonary embolism. Minor arrhythmias are not infrequent when the catheter tip traverses the right ventricle. Ventricular fibrillation has been reported, as has refractory shock. Electrocardiographic monitoring and immediate availability of defibrillating equipment are mandatory. Reactions to contrast material are most frequently minor and primarily consist of urticaria and vasovagal responses. However, patients can occasionally suffer major reactions, with bronchospasm and cardiopulmonary collapse. Renal failure after administration of iodinated contrast material is a well-recognized complication, and preexisting renal disease manifested by elevated serum creatinine levels represents a relative contraindication to angiography. In all circumstances, the amount of contrast material injected should be the minimum required to produce diagnostic opacification of the pulmonary vessels. The use of nonionic or low-osmolar contrast material diminishes the number of minor reactions and increases patient comfort during the procedure. It is now routine in most practices.
Aortography and Bronchial Angiography
Aortography is generally accomplished by the retrograde passage of a catheter from the femoral artery to the aorta or its branches after percutaneous insertion ( eFig. 18-17 ). The study of the aorta and its branches plays a limited role in diseases of the lung. The imaging modalities of choice for diagnosing suspected aortic abnormalities, including aortic dissection, are CT, MRI, and, in acute cases, transesophageal ultrasonography; aortography is seldom required. The diagnosis of pulmonary sequestration once depended on the demonstration of anomalous systemic blood supply by aortography but, in modern practice, chest CT aortography and magnetic resonance angiography (MRA) are often diagnostic and easily demonstrate the abnormal vascular supply ( eFig. 18-18 ). Patients with severe hemoptysis may require bronchial arteriography and embolization of the bronchial artery or arteries supplying the bleeding site ( eFig. 18-19 ). These studies require selective catheterization of the bronchial arteries. Angiographic technique is, for the most part, individualized to fit the particular clinical condition being studied.
Angiography can be performed with a technique termed “digital subtraction angiography.” With digital subtraction angiography, an early image from the angiogram is recorded with an image intensifier and a high-resolution television camera and is digitized and stored. A later image in which vessels are opacified is handled in a similar manner. The first image is then subtracted from the second, and the resulting image is displayed. The background body structures are subtracted, leaving an image of the contrast-filled vessel (see eFigs. 18-17 and 18-19 ). Because the body background does not obscure the final image, angiography can be accomplished with smaller amounts of contrast than otherwise necessary. Motion of structures in the area being studied may make subtraction of images difficult.
Ultrasonography
Ultrasonography, which is also discussed in Chapter 20 , has limited usefulness in thoracic imaging because the ultrasound beam is reflected at the air-soft-tissue interface around the lungs, but some specific uses have been recognized. It is useful for studying vascular, cardiac, and some mediastinal abnormalities, for the localization of pleural fluid and air collections, and for directing interventions (see Fig. 19-3 ). Transesophageal sonography can allow imaging of some mediastinal structures and is often performed to assess the thoracic aorta and heart. The use of ultrasonography in lung imaging is largely limited to the pleura and immediate subpleural areas or to areas of consolidated lung or masses contacting (see Fig. 19-3 ) or invading the chest wall. It is most commonly utilized for the detection, localization, and characterization of pleural effusions and to guide thoracentesis ( eFig. 18-20 ).
Ultrasonography is also valuable in differentiating effusion from pleural thickening. Effusion is usually anechoic or hypoechoic, whereas pleural thickening results in an echogenic stripe within the enclosing rib margin. When free-flowing or layering fluid is not demonstrated on decubitus radiographs in the presence of a thickened pleural stripe, ultrasound study may show collections of loculated fluid surrounded by pleural adhesions. Septations may be visualized within pleural collections, and their shape may change during the breathing cycle, confirming the presence of low-viscosity fluid. A complex echogenic fluid collection indicates the presence of exudate, empyema, or hemothorax, but an echo-free collection does not exclude these diagnoses. Ultrasound is also effective in differentiating subphrenic fluid from pleural effusion because the two hemidiaphragms are excellent reflectors of the ultrasound beam and therefore provide a visible boundary distinguishing intrathoracic from subdiaphragmatic spaces ( eFig. 18-21 ). The presence of a hypoechoic area caudal to the echogenic diaphragmatic stripe but cranial to the liver or spleen indicates ascites.
Ultrasound-guided thoracentesis is frequently performed when loculated fluid is suspected or after attempts at thoracentesis have failed. The use of real-time equipment permits the performance of this procedure at the bedside (see eFig. 18-20 ). After locating the apparent fluid collection, the appropriate depth to which the needle should be inserted is displayed on the screen, and the aspiration is performed in the usual manner. Placement of catheters for drainage can be accomplished in a similar fashion.
Pleural neoplasms, mediastinal masses, or parenchymal masses that abut the pleural surface may be biopsied under ultrasonic guidance (see Fig. 19-3 ), provided that no aerated lung intervenes between the lesion and the pleura. The tip of the needle can be guided and identified within the mass, thus confirming appropriate placement before aspiration or biopsy. However, CT (see Fig. 19-1 , Fig. 19-2 , Fig. 19-6 ) or fluoroscopic guidance is more frequently employed for this purpose.
Computed Tomography
Physical Principles
CT is based on the precise measurement of attenuation of a thinly collimated x-ray beam. Differential x-ray beam attenuation by different tissues forms the basis of image contrast on CT images. The advantages of CT are its axial tomographic (“slicelike”) format and its high sensitivity to differences in density between different tissues.
To obtain CT images, x-rays—produced by a modified standard x-ray tube—are passed through the patient, and the transmitted photons are measured by a series of x-ray detectors. The detectors produce an electric current that is proportional to the intensity of the incident x-ray beam. The strength of this current is measured and digitized by an analog-to-digital converter and thus is available for computer manipulation. The reduction in the intensity of the beam as it passes through the patient’s body is termed “attenuation” and is due to scattering and absorption of the x-ray photons by tissue. The attenuation (more precisely, the linear attenuation coefficient) of each point within the body can be calculated by the scanner’s computer, provided that multiple measurements of x-ray attenuation can be made from different angles. The details of the x-ray beam and detector motion by which these multiple measurements are obtained vary considerably among scanners.
Spiral, or helical, CT scanners, and more recently, multislice CT scanners, use a continuously rotating gantry (containing the tube and detector array) and are capable of imaging the entire thorax in a single breath-hold.
Because the x-ray attenuation measurements are stored in the computer, the image can be enhanced or manipulated mathematically with specific reconstruction algorithms. For example, the technique of high resolution CT (HRCT) combines narrow collimation and image reconstruction with a high-spatial-frequency algorithm to produce increased sharpness in the final stage.
Image Display
The reconstructed CT image is made up of a matrix of picture elements or “pixels.” The x-ray attenuation of each pixel is normalized to that of a test object containing pure water. The CT number is the ratio of tissue attenuation minus water attenuation relative to water attenuation multiplied by 1000 and is expressed in Hounsfield units (HU). The CT number for normal lung ranges from −700 to −900 HU, whereas soft tissues have CT numbers ranging from −100 (fat) to +100 HU (blood clot), with water measuring 0 HU and most soft tissues in the 20 to 60 HU range. The range of CT numbers encountered in patients is approximately 2000, ranging from −1000 (air) to +1000 (bone). However, although the computed image contains 2000 number levels, which could correspond to 2000 shades of gray, the human eye can perceive only about 16 to 20 distinct shades of gray. It is thus necessary when displaying CT images to restrict the image display to a small fraction of the actual range of attenuation values.
This restriction is done first by combining similar CT numbers into a single gray shade and second by setting the window width and window level display settings. The window width is the range of densities that will be displayed as shades of gray; all higher pixel values are shown as white, and all lower ones are shown as black. The window level is simply the median pixel value about which the display range is centered. Thus, to view the lungs, an appropriate window level is −700 HU, with a window width of approximately 1200 HU, which may be adjusted depending on user preference. For viewing soft tissues, pleural space, mediastinum, or hila, a window level of 20 to 40 HU with a width of approximately 400 HU is preferred. These are usually referred to, respectively, as “lung window” and “mediastinal or soft-tissue window” settings, and both should be displayed for a chest CT study.
Spiral and Multislice Computed Tomography
The terms “spiral” and “helical” CT are essentially equivalent; they refer to the path the rotating x-ray beam describes as a result of its continuous rotation around the longitudinal axis of the patient as the patient is transported though the CT gantry on a continuously moving table. In many patients, the entire thorax can be scanned during a single breath-hold. Spiral CT has the advantages of (1) volumetric imaging, which ensures contiguous image reconstruction and allows multiplanar or three-dimensional reconstructions to be performed; (2) more rapid scanning; and (3) more rapid contrast infusion with denser opacification of vessels.
Volumetric CT refers to the ability of modern CT scanners to acquire scan data continuously between two points (in the thorax, typically from the cervico-thoracic junction to the diaphragm), rather than, as with older CT technology, using a “stop-and-shoot” method. With the latter technique, individual image “slices” could be summed to create a volume of imaged tissue but were limited by misregistration between the individual image slices, particularly between successive individual breathholds. Owing to the rapid acquisition times of modern CT scanners, the entire thorax can now be imaged completely in a single breathhold, leading to a single “block” of tissue data that can be reconstructed into practically any desired slice thickness and imaging plane desired.
Multislice CT (MSCT) markedly improves on the advantages offered by single-slice spiral CT. MSCT scanners acquire information using multiple channels during a single tube rotation, thereby dramatically increasing the data acquisition rate. Current MSCT scanners may acquire up to 320 images per tube rotation for a given collimation, whereas routine single-slice spiral CT scanners would only acquire 1 image per tube rotation for a similar collimation. The speed advantage of MSCT scanning is obvious, and this tremendous speed allows for rapid imaging of large volumes of tissue in practically any phase of intravenous contrast enhancement. The image data sets provided by MSCT scanners also allow for improved-quality reformatted images ( eFig. 18-22 , and ). Furthermore, the imaging data acquired with MSCT scanning allow for “retrospective reconstruction” of narrow section images (e.g., “thin sections” or “high-resolution” images) following scan completion.
With MSCT, the ability to reconstruct narrow section images retrospectively has transformed the process of nodule characterization. In the era of single-slice CT scanning, when narrow section imaging was used to characterize a pulmonary nodule, particularly by the detection of calcium or fat within the nodule, either the patient would be held within the department and the radiologist contacted to review the scan to determine if narrow section imaging was required (with the associated detrimental effect on departmental throughout), or the patient would be called back at a later time for further imaging (with the associated patient and referring physician inconvenience). With MSCT scanning, CT protocols that acquire narrow sections (often on the order of 1 mm or less) are devised but are reconstructed using wider sections (often 5 mm) for routine interpretation, which allows for optimized computer performance and data handling; however, the narrow sections have been acquired and are available for review, if needed. In the foregoing example, if narrow sections are needed for pulmonary nodule characterization, the narrow section data may be retrospectively reconstructed and reviewed ( Fig. 18-10 ). In fact, many radiology departments prospectively reconstruct both wider section widths for routine viewing and narrow section widths for specialized applications and save both series to the picture archiving communication system; such an approach strikes an excellent balance between the need for smaller data sets to facilitate routine data handling, while still allowing for specific questions to be addressed later through the use of thin-section imaging without reimaging the patient.

Computed Tomography Scan Protocols
CT scans are obtained with different parameters, depending on the indication for the examination ( Table 18-1 ). Variables include scanning range, patient position, scan section thickness, table transport speed/pitch, and intravenous contrast injection rate, timing, and volume. These parameters are briefly reviewed later. The influence of table transport speed/pitch on CT protocols has evolved with the proliferation of MSCT scanners. Consideration of these variables is more relevant to cardiovascular imaging and is not discussed here. The interested reader is referred to excellent reviews on the topic of MSCT scan parameters and the technical aspects of MSCT scanning. Owing to MSCT’s rapid scan capability and ability to retrospectively reconstruct narrow section data, in recent years there has been some degree of CT protocol “simplification” compared with the single-slice CT era. For example, single-slice high-resolution CT protocols previously included noncontiguous supine and prone 1-mm inspiratory scans, as well as noncontiguous supine expiratory scanning. Using such a protocol, it was not uncommon to mistake a pulmonary vessel for a lung nodule; the inability to review adjacent sections immediately cranial and caudal to the vessel, owing to the noncontiguous nature of single-slice high-resolution CT protocols, often resulted in a pulmonary vessel simulating a nodule. This high-resolution CT protocol was distinct from the “routine” single-slice CT chest protocol, employing contiguous imaging, that was required to clarify the nature of this potential abnormality. Current MSCT high-resolution CT protocols, however, employ contiguous 1-mm supine imaging routinely, supplemented with noncontiguous 1-mm prone inspiratory and supine expiratory imaging, thus alleviating the difficulties associated with noncontiguous imaging techniques while simultaneously maintaining the benefits of thin-section imaging in the characterization of diffuse lung diseases.
Scan Protocol | Intravenous (IV) Contrast Use? | Common Indications | Notes |
---|---|---|---|
“Routine” chest CT * (including spiral and multislice technology) | Possibly, depending on specific application | Initial and repeat cancer staging (may be performed with IV contrast, depending on tumor type), nonspecific chest pain, cough, or shortness of breath, pleural disease, mediastinal or chest wall mass evaluation, suspected thoracic lymphadenopathy, investigation of focal chest radiographic abnormalities, suspected infection, lung cancer screening | IV contrast injection often beneficial for suspected lymphadenopathy, pleural disease, bronchogenic malignancy staging. Employed for fever of unknown origin (often when chest radiography is unrevealing, particularly for immunosuppressed patients). Often used for evaluation before solid or hollow organ transplant to assess for asymptomatic findings that could represent malignancy. Aggressive radiation dose reduction frequently employed for lung cancer screening studies |
CT pulmonary angiography | Yes | Assessment of suspected acute or chronic thromboembolic disease, pulmonary artery aneurysm, or pulmonary artery anomaly | IV contrast injection timing scaled for optimal pulmonary artery enhancement |
CT aortography | Yes | Assessment of suspected aortic dissection or aneurysm | IV contrast injection timing scaled for optimal aortic enhancement |
Coronary calcium scoring | No | Evaluation for presence of calcified coronary artery atherosclerosis for heart disease risk stratification | Volume of acquisition limited to heart |
Coronary CT angiography | Yes | Evaluation for coronary artery atherosclerosis, aneurysm, or anomaly | Scan performed with ECG gating for motion-free images. Volume of acquisition typically limited to heart |
Cardiac CT | Yes | Evaluation for congenital or acquired structural cardiac disease, pericardial disease, or assessment of specific mediastinal or lung pathology impact on heart | Scan performed with ECG gating for motion-free images. Volume of acquisition typically extended slightly beyond heart to include great vessel anatomy. Contrast injection timing may be adjusted to suit particular indications |
Pulmonary vein CT | Yes | Assessment for pulmonary venous and left atrial anatomy before electrophysiologic ablation | Contrast injection timing optimized for left heart enhancement |
High-resolution CT | Typically not required, although in the case of initial evaluation of suspected sarcoidosis, may be of benefit | Diffuse lung disease at chest radiography or suspected by clinical evaluation—initial diagnostic assessment, biopsy site selection, serial evaluation of treatment efficacy; small airway diseases, bronchiectasis, chronic dyspnea or progressive exercise intolerance, assessment of abnormal pulmonary functions testing, lung transplantation evaluation | Thin-section imaging with prone and postexpiratory imaging as well |
Contrast-enhanced chest CT for solitary nodule evaluation | Yes | Evaluation of an indeterminate solitary pulmonary nodule (typically > 1 cm, <3 cm) | Specific, weight-based contrast injection protocol with structured timing of image acquisition to assess for nodule enhancement. Lack of nodule enhancement strongly suggests an indeterminate nodule is benign |
* “Routine” chest CT section thickness (collimation) varies depending on scanner manufacturer, but typically approximates 5 mm. Narrower sections are routinely automatically obtained with modern multislice CT scanning protocols (on the order of ≤1 mm); however, these images may or may not be reconstructed and archived in the picture archive and communications system (PACS), depending on individual radiology departmental protocols. Even when such images are routinely reconstructed and archived to PACS, often an additional series of images employing a wider reconstruction interval (typically approximately 5 mm) is saved to PACS to facilitate day-to-day interpretation and data handling and storage efficiency.
Scanning Range
In most instances, a chest CT study should encompass the entire thorax, from the lung apices to the posterior costophrenic angles. This is easily accomplished in a matter of a few seconds with MSCT. In patients with lung cancer, chest CT done for staging purposes may include the adrenal glands and liver, although the liver acquisition should be timed properly to obtain images in the correct phase of liver enhancement. In practice, lung cancer staging studies are typically performed as dedicated examinations of the entire chest, abdomen, and pelvis.
Patient Position
Normally, patients are scanned in the supine position. However, decubitus positioning or prone positioning may be used to elucidate whether pleural fluid collections are free or loculated, to distinguish dependent atelectasis from parenchymal fibrosis, or to position lung lesions optimally for biopsy. Images are normally obtained at total lung capacity to obtain maximum air-tissue contrast and to spread anatomic structures and lesions over a larger area, thus minimizing volume averaging. Prone images are usually obtained with HRCT studies. Technical advances now permit the acquisition of CT images during, rather than after, forced expiratory vital capacity maneuvers (see to ).
Scan Section Thickness
In the era of single-slice spiral CT, the thickness of an individual CT section was set to a predetermined value before scanning by setting the x-ray beam to a certain thickness: a process referred to as “collimation.” Typical slice thicknesses for single-slice spiral CT ranged from 1 to 10 mm, with 5- to 7-mm collimation employed for most “routine” chest CT applications. With the development of MSCT systems, although various collimation choices are still possible, most MSCT protocols employ certain detector configurations that either favor narrow detector width (to promote optimal spatial resolution) or wider detector widths (to facilitate rapid volume of coverage). As systems with greater numbers of detectors are developed, the choice of high spatial resolution at the expense of volume of coverage and total length of exposure—an age-old compromise in the era of single-slice spiral CT—is becoming less of a quandary. Modern MSCT systems now allow the entire thorax to be scanned with a narrow detector configuration (1 mm) in a matter of a few seconds. Once such images are acquired, they may be combined in various ways for optimal viewing. For example, a chest CT study obtained on an MSCT system with detector elements arranged in a manner resulting in 1-mm detector configuration would produce several hundred 1-mm images for the thorax of an averaged-sized patient. However, these 1-mm images can be “combined” to generate 5-mm images, and these images can be displayed for routine review; this results in about 50 to 70 images for a typical chest CT. Should the radiologist desire to view the high-resolution, 1-mm images, these images can be reconstructed from the original dataset as long as the original scan data are saved. For example, with single-slice spiral CT scanners, frequently the patient would need to return for additional thin-section (narrow-collimation) imaging to characterize any nodules encountered at the examination. The only way to avoid bringing patients back in this setting was to keep the patient on the scanner while the study was reviewed, but this approach seriously decreases scanner throughput. Even if the patient were held during the scan review, additional nodule characterization with single-slice CT would require repeat scanning with narrow collimation to detect fat or calcium within the nodule, which requires additional radiation exposure. With MSCT, when a nodule is recognized on a study, images may be reconstructed according to the narrowest detector configuration to accomplish this goal long after the patient has left the scanner, without the need for additional scanning.
An additional benefit realized by the development of MSCT is the ability to image using isotropic voxels. The term isotropic voxel indicates that the resolution of the scan in the x-, y-, and z-planes is equal. In other words, MSCT scanning using isotropic voxels allows the CT data to be reconstructed in any plane with resolution equal to that of the axial scans. Most single-slice CT examinations did not possess this capability, and therefore nonaxial reformatted images suffered from degraded quality compared with that of the axial scans. MSCT’s ability to scan using isotropic voxels allows creation of images in any desired plane that are equal in resolution to the axial scans (see eFig. 18-22 , and )—this multiplanar capability was previously an advantage enjoyed by MRI and ultrasound over CT.
Contrast Enhancement
The injection of intravenous iodinated contrast material during chest CT is important in many situations. Administration of contrast material is not routine except for the diagnosis of vascular abnormalities such as aortic dissection, aneurysm, pulmonary embolism, when using a specific protocol in selected patients with pulmonary nodules, or for examinations performed for lung cancer staging or assessment of pleural of chest wall diseases. If possible, iodinated contrast materials are avoided because they are expensive and associated with a small but definite risk of serious reactions and toxicity. Although contrast infusion can be helpful in distinguishing vessels and soft-tissue masses in the mediastinum and hila, it is not considered necessary for diagnosis by all radiologists. For routine CT of the lungs (e.g., to rule out metastases), contrast material is rarely required.
Nonionic and low-osmolar contrast materials have proved to have a lower incidence of adverse reactions than high-osmolar agents. Patients with a prior history of contrast reaction should be treated with extreme care because they are at higher risk for subsequent serious reactions, particularly those rare patients that have experienced contrast reactions despite proper premedication with corticosteroids and antihistamines. If vascular pathology is of interest in such patients, MRI is the preferred modality. If CT with contrast enhancement is necessary, pretreatment with corticosteroids is advisable. Iodinated contrast media reactions (“radiographic contrast-medium-induced leukostasis”) is discussed in Chapter 71 .
The technique of intravenous contrast material administration is important. Peak opacification of vascular structures is desirable. To achieve this, a bolus of contrast material must be administered, preferably via a calibrated mechanical injector, and the area of interest must be scanned rapidly during the initial transit of the contrast agent. Timing of the delivery of intravenous contrast should be adjusted to the organ system of interest. For example, chest CT protocols designed for the detection of pulmonary embolism generally use rather short delays from the time of the beginning of contrast injection to the start of imaging (usually ≤ 20 sec), whereas chest CT protocols designed for the evaluation of aortic dissection generally use slightly longer contrast injection delays.
Medical Imaging and Radiation
The major drawback of the increased utilization of CT is radiation exposure. In 1980–1982, radiation from medical imaging accounted for approximately 18% of the per-capita effective radiation dose in the United States, but this value increased to 54% by 2006, due largely to increased radiation doses resulting from CT scanning and, to a much lesser extent, nuclear medicine procedures. It was recently reported that CT represents about 17% of all radiologic procedures in the world but accounts for more than 40% of the collective dose. Given the continued increased use of CT since 2006, it is likely that these numbers are now even greater.
The two primary terms for describing the radiation imparted by a CT scan are adsorbed and effective dose. The adsorbed dose, measured in grays (Gy), is the energy absorbed per unit mass of a specific organ. In other words, it is the radiation imparted to a specific organ and thus accounts for the future risk of developing a malignancy at that site. Effective dose, measured in sieverts (Sv), is an overall estimate of total-body radiation exposure; this unit attempts to provide a measure of the overall potential harm caused by radiation exposure. Effective dose is defined as the radiation dose that must be delivered to the whole body to yield the same biologic consequences (specific for cancer induction only) as the dose actually received by the exposed organs. This is calculated by summing the individual adsorbed doses to each organ, each of which is multiplied by a weighting factor reflecting individual organ radiosensitivities. Effective dose is useful for gross comparisons between different modalities and techniques. It should be understood that effective dose is not an actual measure of the radiation imparted to a patient during a given CT examination, although many published articles use this unit (often expressed as milliSieverts [mSv] or in the form of the older unit, millirems [mrem]) in this fashion. This misconception is compounded by the recording of a “patient dose report” as part of the CT scan report ( eFig. 18-23 ). Rather, the actual radiation dose imparted to a patient during a CT examination depends directly on the size and shape of the actual patient. The units reported by the CT scanner, often the CT Dose Index (usually expressed as the CTDI vol , reported as an absorbed dose, in mGy) or the Dose-Length Product (DLP, reported as an effective dose, in mSv, and equal to the CTDI vol multiplied by the irradiated scan length), are measures of scanner radiation output, not patient dose. Calculating the radiation dose imparted to a patient during a given CT scan requires complex equations that take into account patient size, shape, body composition, and the organs irradiated, as well as scanning range. With knowledge of the patient size, body region scanned, CT scanner output, and scan length, a reasonable approximation of patient dose for a given CT scan is possible, but it remains imperative to understand that the units of scanner output—the CTDI and DLP—are not measures of patient radiation dose. Therefore, these parameters should not be used to estimate potential cancer risk or radiation-related deterministic effects (e.g., skin injury) for individual patients undergoing medical imaging procedures.
Approximate effective doses (scanner outputs) for CT are given in Table 18-2 and compared with nonmedical radiation exposures. Of note, the values given are representative measurements but, in fact, there is a wide range of exposures dependent on CT technique and method of estimation. Also, indications such as pulmonary embolism, which require narrower sections, may involve a relatively greater amount of radiation exposure compared with “standard” chest CT protocols. In recent years, concerted efforts to curtail medical radiation exposure have resulted in a number of advances that have significantly reduced the amount of radiation delivered to the patient, chiefly through reduction of CT-related exposures. A thorough review of these methods is beyond the scope of this brief discussion but includes increasing public and provider awareness through efforts such as the “ImageGently” campaign conducted by the Alliance for Radiation in Safety in Pediatric Imaging and the ACR’s “ImageWisely” (radiation safety in adult medical imaging) effort; technical advances in CT scanner equipment (new reconstruction algorithms and automated exposure controls that more efficiently apply the radiation exposure to the patient, such as automatically reducing tube current through thinner body regions); encouraging the use nonionizing radiation imaging alternatives for selected disorders; and active interventions by radiologists, including limiting scanning range and reducing x-ray tube current and voltage, among other considerations. It is now relatively commonplace that exposures associated with newer CT protocols are significantly less than the values reported in Table 18-2 ( ).
Radiation Exposure | Effective Dose (mSv) |
---|---|
U.S. annual per-capita effective radiation dose 2006 (background/medical/total) | 2.4/3.0/5.6 |
Posteroanterior chest radiograph * | 0.02 |
Ventilation/perfusion scan * | 1.4–2 |
Standard chest CT | 5–8 |
“Low-dose” chest CT | 2 |
High-resolution chest CT (1-cm noncontiguous intervals) * | 1 |
Catheter pulmonary angiography * | 2.3–4.1 |
Whole-body PET * | 14 |
Coast-to-coast U.S. flight (≈3000 miles) † | 0.03 |
Living near coal-fired power plant † | 0.0003 |
Proximity to x-ray luggage inspectors | 0.00002 |
Living within 50 miles of a nuclear power plant † | 0.00009 |
Living in Denver for 1 year | 1.8 |
Airport backscatter device | 0.000015–0.00088 |
Smoking, 1 year ‡ | 2.8 |
* Values may vary according to individual equipment manufacturers, patient size, duration of study, use of dose-reduction techniques, and individual institutional protocols.
† Data from http://www.ans.org/pi/resources/dosechart/
‡ Data from http://web.princeton.edu/sites/ehs/osradtraining/backgroundradiation/background.htm
Considerable controversy regarding the genesis and magnitude of adverse effects resulting from radiation exposure in humans exists and has been the subject of debate for decades. The rapid rise in the use of CT scanning in recent years, as well as several well-publicized patient overexposure events at CT scanning, have fueled this debate. Nevertheless, several points can be agreed on by investigators on both sides of this debate:
- 1.
Radiation is potentially harmful to biologic systems and exposure can induce cancer, among its other effects ;
- 2.
There is relatively clear evidence for radiation-induced carcinogenesis for exposures exceeding 100 mSv ;
- 3.
Radiation is a relatively weak carcinogen, and excess radiogenic cancer risk, if any, is considerably less than spontaneous cancer risk ;
- 4.
There are no parameters that allow a radiation-induced malignancy to be distinguished from a “naturally occurring” one ;
- 5.
The risk of radiation-induced malignancy is generally inversely related to age and relatively higher in women compared with men ;
- 6.
The risk for radiation-induced carcinogenesis from a CT scan is generally far less than the benefit of the information gained from appropriately indicated CT studies.
The primary source of controversy between those who believe low-level radiation exposures—exposures less than 100 mSv, which are typical for the exposures associated with medical imaging—may result in the development of malignancy and those who claim no such evidence exists lies with the linear nonthreshold (LNT) model for radiation-induced carcinogenesis. The LNT theory holds that a straight-line relationship between radiation dose and cancer risk exists, and there is no threshold dose below which radiation is not carcinogenic. Proponents of the LNT theory hold that a linear model best describes the relationship between radiation exposure and cancer induction, and no evidence to support a departure from the LNT model exists ; by contrast, critics of the LNT model for radiation-induced carcinogenesis risk prediction insist that the LNT model was developed to establish standards for protection of occupationally exposed individuals and overestimates the incremental risk for cancer development at low-level radiation exposures, and, therefore, it is inappropriate to project individual cancer risks from medical radiation exposures using this model. Nevertheless, many of the widely publicized reports estimating the number of cancer-related deaths resulting from CT scanning have used the LNT methodology.
Estimates for the risk of radiation-induced carcinogenesis in humans primarily stem from epidemiologic studies of exposed patient cohorts, particularly Japanese survivors of the atomic bombings in 1945; other data sources include occupational radiation exposures and radiation accidents, such as the Three Mile Island and Chernobyl accidents. The LNT model is often used to extrapolate the elevated rate of malignancy found among these exposed cohorts to patients receiving the relatively low-level radiation exposures, typical of medical imaging, in the effort to estimate individual cancer risks; in addition, both the National Council on Radiation Protection and Measurements and the International Commission on Radiological Protection advise against the use of the effective dose paradigm and LNT theory in this fashion. Furthremore, it should be understood that the LNT model does not provide for the ability to “sum” separate radiation exposures from temporally separated radiation-utilizing procedures into a “cumulative” cancer risk. In other words, regardless of the patient’s previous radiation exposures, the imaging study the patient is about to undergo only adds the potential incremental risk for cancer development attributable to that examination ; the radiation exposures from separate examinations are not additive. This notion carries significant implications regarding the recent trend toward the development of radiation “dose registries,” whereby patient medical imaging radiation exposures are tracked in a database with the intention of using such information to make future medical imaging decisions.
Low-Level Radiation-Induced Malignancy: Evidence for and Against
Investigators who assert that low-level radiation has carcinogenic potential cite the elevated incidence of malignancy noted in many large epidemiologic cohorts of exposed patients, particularly the Japanese atomic bomb survivors in the Life Span Study. This argument relies on the LNT model to extrapolate the experience of these cohorts, exposed at relatively higher rates, to estimate the risks associated with the lower-level radiation exposures typical of medical imaging. These investigators assert that a linear relationship (e.g., the LNT model) best describes the relationship between cancer rates and radiation exposures over a wide range of exposures; moreover, this relationship is consistently observed not only in the Life Span Study but in the analysis of other large epidemiologic studies of individuals exposed to radiation, and that linearity best describes the responses of biologic systems to radiation exposure. Furthermore, experimental evidence can directly show cellular damage induced by radiation. Finally, a recent large epidemiologic study focusing on younger patients undergoing CT scanning has shown a significant linear association between radiation dose to the brain and bone marrow and subsequent development of brain tumors and leukemia, respectively. This study evaluated 180,000 patients younger than 22 years undergoing nearly 280,000 CT scans in the United Kingdom between 1985 and 2002, who were subsequently followed for cancer development; the investigation specifically focused on brain tumors and leukemias, which are the cancers expected to appear first in irradiated pediatric patients ; the risks for brain tumor and leukemia development in this patient cohort were small: it was estimated that one head CT may result in one excess brain tumor, for every 10,000 patients undergoing CT, in the the first decade following the exposure. However, a recent editorial also noted that the relatively short follow-up time in this study (average 10 years) is insufficient to detect all potentially radiation-induced malignancies in this patient cohort, given that the latency of some radiation-induced malignancies may be as long as 20 to 40 years. Extrapolating the 10-year risk for radiation-induced carcinogenesis for the patients studied to lifetime carcinogenic risks for the exposed individuals yields estimates for cancer induction that are roughly similar to those asserted by the Life Span Study. Note that this study focused on pediatric patients, in whom the individual risks for radiation-induced malignancy are higher than for adults, but more than 90% of CT scans are performed in adult patients.
Investigators who challenge the notion that low-level radiation poses significant carcinogenic potential note the difficulties resulting from the reliance on the LNT model, as discussed previously, but also point to significant differences among the patients in the Life Span Study compared with those undergoing procedures utilizing medical radiation. Such differences include the following: that cancer incidence in Japan today is substantially different than in the United States, and that cancer incidence in both countries today is likely substantially different than in Japan in 1945. Medical imaging radiation exposure sources include relatively low energy x-rays and gamma rays, whereas atombic bomb explosions exposed patients to high-energy gamma rays, neutrons, and other charged particles ; medical imaging typically employs fractionated doses (radiation doses delivered intermittently over a period of time), whereas atomic bomb blasts exposures are instantaneous ; and Japanese atomic bomb survivors suffered uniform, whole-body exposures and radioactive fallout, whereas patients underoing medical-imaging procedures typically only expose limited body regions in a nonuniform fashion (the exception being some nuclear medicine procedures). Furthermore, the health of Japanese atomic bomb survivors was comparatively compromised, compared with patients undergoing medical imaging, owing to the limited resources for medical care, food, and shelter in Japan following the atomic bomb blasts. Thus, it seems clear that these substantial population differences limit the ability to extrapolate radiation-induced cancer incidence from one cohort to another.
In contrast to other investigators, those who challenge the belief that low-level radiation has carcinogenic potential assert that the results of epidemiologic studies of radiation-induced carcinogenesis show no, or much smaller, health effects from radiation exposure than the data from the Life Span Study. Additionally, these investigators have called attention to serious limitations in the manner in which radiation-induced malignancy rates are calculated through the generation of “lifetime attributable risk” modeling.
Lines of biologic evidence also call into question the simple notion that the rate of cancer induction is proportional to the amount of radiation exposure. For example, it has been suggested that a 0.1 Sv radiation dose, which is at the upper limit of what is referred to as “low-level radiation,” is estimated to cause 0.004 long-term mutations per cell, which is a minor addition to the 1 mutation per cell per day that results from natural processes. Furthermore, a number of in vivo experiments have shown a reduced rate of chromosomal aberrations following low-level radiation exposure due to stimulated production of DNA repair enzymes and immune system activation—the so-called “adaptive response.” Finally, the same survival curves plotted for Japanese atomic bomb survivors and other large-scale epidemiologic investigations of radiation effects, interpreted by some investigators as supporting the notion of a linear-dose response relationship between radiation exposure and carcinogenesis, are interpreted by others as showing a threshold effect, below which cancer is not induced, or in some cases, below which cancer risk actually decreases. The latter concept has been referred to as “hormesis” and is thought to be due to upregulation of protective mechanisms within cells and tissues induced by low-level radiation.
Although conflicting interpretations are difficult to reconcile and data on both sides of the argument can be compelling, in practice, employing medical radiation only when necessary and substituting nonradiation utilizing procedures and dose-saving methodologies whenever possible should provide an optimal approach to balancing the immediate need for diagnostic information with patient safety.
Magnetic Resonance Imaging
Physical Principles
MRI is performed by magnetizing the patient’s tissue slightly, generating a weak electromagnetic signal by applying a radiofrequency pulse, and mapping that signal spatially by manipulating its frequency and phase in a location-dependent manner with magnetic field gradients. MRI does not require mechanical motions of the scanner and therefore can image directly in nonaxial planes.
Although MRI uses electromagnetic radiation, the energy levels used in MRI are quite low (i.e., nonionizing). MRI appears to be remarkably free of significant bioeffects. Potential safety hazards in MRI relate to the extremely strong static magnetic field and rapidly switched gradient magnetic fields used and to the possibility of tissue heating from radiofrequency energy absorbed by the body. Tissue heating is a theoretic concern but, in practice, is not significant when conventional MRI techniques are used. Theoretically, the rapidly varying gradient magnetic fields could stimulate electrically excitable tissue, but this is not a problem with routine MRI techniques. In contrast, the powerful static magnetic field is a major safety hazard because the magnetic forces near a whole-body magnetic resonance (MR) imager are strong enough to cause significant projectile hazards. For example, an ordinary steel oxygen cylinder brought into an MRI examination room will fly into the bore of the magnet with a terminal velocity of about 45 mph. The possibility of displacements or torques on metallic implants within patients also must be considered. Although the margin of safety is high, there are rare documented instances of harm to patients from dislodging intracranial aneurysm clips or intraocular metallic foreign bodies. Finally, the magnetic field can operate reed relays in cardiac pacemakers and cause a change in the pacing mode. Accordingly, strict security around MRI facilities is essential to prevent patients with certain types of metallic implants from entering the scanner and to prevent medical personnel from carrying into the scan room objects that could become projectiles.
MRI produces extremely high contrast between different types of soft tissue. This soft-tissue contrast is based on intrinsic properties of the tissues, but it can be modified and exploited by appropriate use of operator-selectable imaging techniques. The tissue properties normally utilized in MRI are as follows: the concentration of protons available to produce an MR signal (“proton density”), the presence of motion or blood flow, and two properties known as T1 and T2, time constants that describe how quickly an MR signal can be generated from a tissue (T1) and how quickly the MR signal, once generated, decays away (T2). In general, pathologic tissues have long T1 times and appear dark on those MR images whose appearance is conditioned primarily by T1 effects (“T1-weighted images”). Usually, pathologic tissues also have long T2 times and appear bright on T2-weighted images. The reason for this opposite behavior is that “T1” and “T2” describe processes that have opposite effects on the intensity of the MR signal. Flowing blood also can appear either bright or dark on MR images, depending on the examination technique used. The art of performing an MRI examination depends on appropriate manipulations of imaging techniques to capitalize on differences in proton density, flow, T1, and T2 between normal and pathologic tissue. In addition, the use of gadolinium-based MRI contrast materials, which alter the T1 and T2 of tissues semiselectively, has become important for numerous clinical applications, particularly MR angiography (MRA). MRI also can display other tissue properties such as molecular self-diffusion, temperature, and metabolites (MR spectroscopy), but these are less important for thoracic applications, although diffusion imaging and MR spectroscopy have recently shown some promise in lung cancer evaluation and staging.
Techniques
Motion during MRI causes artifacts that can degrade image quality severely. Motion compensation techniques, such as electrocardiographic gating and respiratory compensation, are widely used in chest MRI. High-speed techniques permit imaging in a matter of seconds, even fast enough to stop cardiac motion.
Direct MRI in sagittal, coronal, and oblique planes can provide superior depiction of structures that are oriented in the long axis of the body, such as the aorta, and of edges of structures that lie within the axial plane, such as lesions in the aortopulmonary window or lung apex.
Flow has profound effects on the MR image. Two basic effects from flow are seen in MRI; they are called “time-of-flight effects” and “spin-phase effects.” The manifestations of flow effects in MR images strongly depend on the type of flow that is present and on the specific MRI techniques used. The clinical implication of this is that one can make the MR image of blood either bright (“white-blood images”) or dark (“black-blood images”). Most types of vascular pathology can be demonstrated with either white-blood or black-blood images, but there are usually clinical advantages to using one approach or the other in specific situations. Flow velocity can be estimated with MRI techniques, and thus noninvasive estimates of blood flow are potentially available.
Although high-speed imaging techniques are now available with MRI, it still is not an appropriate method to use for wide-ranging screening examinations of the entire trunk for metastatic disease. Such examinations normally should be performed with modern CT scanners. It usually is more rewarding to ask an anatomically focused question when requesting an MRI study.
Applications of Conventional Chest Radiography
Screening and “Routine” Chest Radiographs
It is now generally agreed that screening chest radiographs are not indicated except in specific high-risk populations. Estimates as to possible iatrogenic disease caused by ionizing radiation from screening examinations, forecasts of possible genetic consequences, and financial concerns outweigh the medical value of such examinations. In 1973, the Department of Health, Education, and Welfare, in conjunction with the ACR and the American College of Chest Physicians, recommended the discontinuation of screening examinations. In 1985, recommendations of the ACR were more explicit: chest radiographs obtained for routine examination, preemployment screening, prenatal or obstetric screening, and hospital admission, as well as repeated examinations of patients with positive tuberculin tests and a negative initial chest radiograph, should be eliminated. From their analysis of more than 10,000 chest radiographic examinations obtained in a hospital-based population, Sagel and associates concluded that routine screening examinations done solely because of hospital admission or scheduled surgery are not warranted in patients younger than 20. Even in selected high-risk populations, radiographic screening for carcinoma of the lung has failed to significantly increase longevity.
The value of the routine hospital admission chest radiograph is still controversial. Although the yield is low in asymptomatic patients, the examination can be extremely valuable as a baseline study for comparison with radiographs taken during or after the course of hospitalization in patients who develop pulmonary symptoms.
Detection of Lung Cancer and Assessment of Solitary Pulmonary Nodules
Although chest radiographs are clearly useful in the initial evaluation of patients suspected of having lung cancer, they are of limited accuracy in showing small or early cancers. At the time of their initial diagnosis, it is not uncommon for lung cancers to be visible retrospectively on prior radiographs. Although additional radiographic studies, such as oblique views, are cost-effective for the initial evaluation of “nodular opacities” (see eFig. 18-13 ) that do not clearly represent a lung nodule and are often of value in determining that a “nodular opacity” is not a true nodule, studies by CT are superior for the detailed evaluation of a known lung nodule.
Evaluation of Intensive Care Unit Patients
Chest radiography is generally the most common imaging study performed in the most critically ill patients in the hospital—those in the ICU. ICU radiographs are taken with portable and, therefore, suboptimal technique; nonetheless, the information they provide is useful and may alter patient management.
The overall incidence of abnormalities found on chest films in ICU patients is quite high; in a study of more than 1000 consecutive medical or surgical ICU films obtained routinely or after a change in clinical status, malposition of a monitoring device or a marked change in apparent cardiopulmonary status was found in 65%. In a study of patients in a respiratory ICU, chest radiographs showed at least one new, clinically unsuspected finding in 35% of patients that, in 29% of these patients, led to a change in management. Significant findings are even more likely when the study is obtained to assess a change in clinical status. In intubated patients in a medical ICU, 43% of radiographs showed significant worsening of a known process or development of a new abnormality. The value of obtaining chest radiographs on a daily basis in ICU patients is less clear. Strain and colleagues found that routine morning radiographs revealed unsuspected abnormalities that led to a change in management in only about 15% of medical ICU patients, although this percentage was considerably higher (57%) in patients with pulmonary or unstable cardiac disease. Graat and coworkers prospectively assessed the value of 2457 routine chest radiographs performed on patients in a combined medical and surgical ICU and found only 5.8% of routine chest radiographs showed new or unexpected findings; only 2.2% of patients required a change in management. Hall and colleagues found that 18% of mechanically ventilated patients in a medical/surgical ICU had at least one radiograph that showed a clinically significant and unsuspected finding. The value of daily chest radiography in patients who have undergone thoracotomy and pulmonary resection has also been challenged. Daily chest radiography in nonhypoxic patients who had recently undergone thoracotomy and pulmonary resection changed care in only 27% of patients, whereas chest radiography altered management in 79% of such patients who were hypoxic. Finally, a meta-analysis of 8 studies totaling 7078 patients found that elimination of daily routine chest radiography did not affect hospital or ICU mortality and there was no difference in length of stay in either the ICU or hospital, or the period required for mechanical ventilation, between patients undergoing routine daily chest radiography and those receiving “on-demand” imaging. Similarly, Hejblum and associates concluded that an on-demand strategy for ordering chest radiographs for mechanically ventilated patients in the ICU is associated with a 32% reduction in the use of chest radiography compared with a routine daily strategy, but both strategies result in a similar rate of therapeutic or diagnostic intervention. On the basis of these results, the ACR has recommended that portable chest radiography should be obtained only for clinical indications, not routine assessment, when monitoring a stable patient or for a patient undergoing mechanical ventilation in the ICU. Furthermore, routine chest radiography is not recommended for stable patients admitted to the ICU for cardiac monitoring or for stable patients admitted to the ICU for extrathoracic disease only. Chest radiography is still recommended for the assessment of patients following initial support device placement, including endotracheal intubation, central venous catheter insertion, pulmonary arterial catheter placement, nasogastric tube placement, and thoracostomy tube insertion.
Indications in Acute Lung Disease
Dyspnea
Two studies suggest that chest radiography should be used routinely in patients with acute or chronic dyspnea. In one study, new, clinically important radiographic abnormalities requiring acute intervention or follow-up evaluation were identified in 35% of 221 symptomatic patients. Another study found that acute dyspnea was a strong predictor of a radiographic abnormality, but mainly in patients older than 40. In this group, 86% of dyspneic patients had abnormal chest radiographs, whereas radiographs were abnormal in only 31% of patients younger than 40. Only 2% of patients younger than 40 with a normal physical examination had abnormal radiographs indicative of an acute abnormality. The ACR recommends chest radiography when dyspnea is chronic or severe or when additional risk factors are present, such as age older than 40; known cardiovascular, pulmonary, or neoplastic disease; or abnormal physical findings.
Acute Respiratory Symptoms
Opinions are also divided about the utility of chest radiographs in patients with suspected acute lung disease and symptoms other than dyspnea. In a study of 1102 outpatients with acute respiratory disease, Benacerraf and coworkers found patient age, results of physical examination, and presence or absence of hemoptysis to be important factors in predicting the value of radiographs; only 4% of patients younger than 40, without hemoptysis and without detectable abnormalities on physical examination, had acute radiographic abnormalities, whereas a much higher incidence of radiographic abnormalities was present if the patient was older than 40, had hemoptysis, or had abnormal physical findings. Heckerling, in a study of 464 patients with acute respiratory symptoms, found a low incidence of pneumonia (3%) in patients with a negative physical examination, except in those with dementia. Whenever pneumonia is suspected in adults, the American Thoracic Society recommends PA (and lateral when possible) chest radiography although the impact of chest radiography on clinical outcomes in patients with lower respiratory tract infections remains unclear. In this setting, chest radiography may be useful to determine which patients should be hospitalized and which patients should be classified as having “severe” pneumonia. The ACR considers chest radiography appropriate in immunocompetent patients with acute respiratory illnesses when one or more of the following is present: patient older than 40; dementia, positive physical examination findings; hemoptysis, hypoxemia, leukocytosis, and a number of other risk factors, including coronary artery disease, congestive heart failure, or drug-induced respiratory failure. For patients younger than 40 with an acute respiratory illness but without such additional findings, chest radiography is generally not routinely indicated. Chest radiography is also considered appropriate for any patient clinically suspected of pneumonia.
Acute Asthma
Chest radiography is uncommonly used to make a diagnosis of asthma; radiographs are often normal, and visible abnormalities in this disease are usually nonspecific. Although Petheram and associates reported that 9% of 117 patients with severe acute asthma had unsuspected radiographic abnormalities affecting management, the usefulness of radiography in both adult and pediatric patients with an established diagnosis of asthma who suffer an acute attack is limited. Correlation between the severity of radiographic findings and the severity or reversibility of an asthma attack is generally poor, and radiographs provide significant information that alters treatment in 5% or fewer patients with acute asthma. Although it is difficult to generalize regarding the role of radiographs in both adults and children with acute asthma, chest imaging should be used to exclude the presence of associated pneumonia or other complications when significant symptoms and/or appropriate clinical or laboratory findings are suggestive. The ACR considers chest radiography warranted in adult patients with asthma when a clinical suspicion for pneumonia or pneumothorax is present, or unless one or more of the following is noted: chest pain, edema, leukocytosis, or the patient has a history of either coronary artery disease or congestive heart failure.
Exacerbation of Chronic Obstructive Pulmonary Disease
Chest radiographs are often used in the initial assessment of patients with suspected COPD; however, they are of limited value in patients with known COPD who present with worsening of their disease. In a study of 107 patients with COPD presenting with an exacerbation of symptoms, only 17 (16%) had an abnormal chest radiograph, and in only half of these did the radiographic findings result in a significant alteration in management. In another study, including patients with both COPD and asthma, the management of 21% of patients was altered by radiographic findings. It has been recommended that chest radiographs be obtained in patients with COPD only if certain clinical indicators are present; in various studies, these have included a clinical suspicion for pneumonia or pneumothorax, a history of coronary artery disease or congestive heart failure, leukocytosis, chest pain, peripheral edema, intravenous drug abuse, fever, seizure, immunosuppression, and other pulmonary disease. When such indicators are used as a guide, nearly all patients with significant findings will have radiographs performed; moreover, it should be noted that more than two thirds of patients in one study met inclusion criteria for performing radiography.
In most patients with an established diagnosis of cystic fibrosis, clinical findings and chest radiographs are often sufficient for clinical management. Conversely, it should be recognized that patients with cystic fibrosis can have a significant exacerbation of their symptoms with little visible radiographic change.
Applications of Cross-Sectional Imaging Techniques
Solitary Pulmonary Nodules
Assessment of a solitary pulmonary nodule (SPN) seen on chest radiographs is a common indication for CT. CT is used to confirm that the SPN is real, to confirm that the SPN is solitary, to attempt further noninvasive characterization of the lesion, to guide percutaneous biopsy of the lesion, and to provide staging information if the SPN is found to represent a carcinoma. The likelihood of malignancy in such nodules varies from less than 10% in mass screenings to about 50% among resected nodules.
In general, CT of a patient with an SPN should be performed with thin-section volumetric imaging to provide detailed analysis of nodule morphology and to allow identification of the presence of fat or calcium (see Fig. 18-10 , ) within the nodule, and when the latter is present, the pattern of calcification; this is now fairly routine in the era of MSCT technology.
In up to 20% of instances, a “lung nodule” visible on chest radiographs actually represents an artifact, chest wall lesion ( eFig. 18-24 ), or pleural abnormality; in some cases, CT is essential to determine the true nature of the opacity. CT can be useful to define the morphology of the SPN and suggest whether it is benign, likely malignant, or indeterminate, in other words, having neither benign nor malignant characteristics. In some patients, a specific diagnosis of lesions such as rounded atelectasis ( Fig. 18-11 , ), a mucous plug, or arteriovenous malformation ( ) can be made on the basis of CT findings, indicating the benign nature of the lesion. Other CT appearances suggest the presence of malignancy ( eFig. 18-25 ). Malignant features include a spiculated (see eFig. 18-25D ) or irregular contour; the presence of air bronchograms within the nodule (see eFig. 18-25A ), bubbly air collections (“pseudocavitation”), or cavities; and a diameter larger than 2 cm ( Fig. 18-12 , see eFig. 18-25A ). Benign features include the presence of several patterns of calcification; “benign” patterns include diffuse, central, laminated, and chondroid, or “popcorn,” calcification ( Fig. 18-13 ). In about 30% of benign nodules, calcium not readily visible on chest radiographs can be seen on thin-section CT ( eFigs. 18-26 and eFigs. 18-27 ). Carcinomas may occasionally show calcification ( eFig. 18-28 ), although often in an eccentric or stippled pattern. The presence of fat within an SPN, indicated by a low CT attenuation coefficient ( Fig. 18-14 ), strongly suggests the presence of hamartoma or lipoid pneumonia ( eFig. 18-29 ); such nodules can be safely followed with serial radiographs.




Malignant tumors tend to show greater enhancement than benign nodules after the rapid injection of iodinated contrast material. Because the degree of enhancement depends on the amount and rapidity of contrast infusion, it is important to use a consistent technique. Using a specific contrast enhancement CT protocol, a threshold for enhancement of 15 HU or more is typically seen with malignancy, hamartoma, and some inflammatory lesions. Enhancement of less than 15 HU almost always indicates a benign lesion, usually a granuloma. Therefore, whereas positive results (enhancement of >15 HU at any time point during the study) are nonspecific, negative results are quite useful ( eFig. 18-30 ). This technique has been shown to have a sensitivity of 98% and a specificity of 58% in diagnosing carcinoma. More importantly, the negative predictive value of this technique is approximately 96%. CT nodule enhancement studies are most appropriately used for patients who have indeterminate nodules (i.e., those without typical benign or malignant appearances). A similar use for dynamic, contrast-enhanced MRI has been reported, although data are limited. The use of fluorodeoxyglucose positron emission tomography (PET) imaging (see Chapter 21 ) has also been shown to be useful for distinguishing benign from malignant nodules (see Fig. 21-1 ) and is generally favored over contrast-enhanced CT for additional imaging characterization of indeterminate lung nodules. In a meta-analysis, PET was shown to be 94% sensitive and 83% specific for the differentiation of benign from malignant solid nodules 1 to 3 cm in size. The addition of PET-CT tends to increase sensitivity with no change in specificity compared with PET alone. False-positive PET results may be seen with inflammatory processes, particularly granulomatous diseases such as tuberculosis (see eFig. 53-6C and D ), histoplasmosis, and sarcoidosis. Tumors that may be 18 PET-negative include minimally invasive adenocarcinoma (see Fig. 21-2 , eFig. 53-7A ), carcinoid tumors, and some metastases (e.g., renal cell carcinoma). In addition, small nodules may not be of a sufficient size to produce a positive result with PET scanning (see eFig. 53-7F ). The sensitivity of PET imaging significantly decreases with nodules measuring less than 8 to 10 mm in diameter (see eFig. 53-7F ). As with any imaging modality, the correlation of the PET results with morphologic features on CT, prior radiographic examinations, and the clinical presentation will improve the determination of the likelihood of malignancy.
Multiple Pulmonary Nodules
CT is the favored procedure for identifying multiple pulmonary nodules or masses. It detects more and smaller metastases ( Fig. 18-15 , ) than any other imaging technique, and 2- to 3-mm nodules are routinely visible. This is most relevant for patients with extrathoracic malignancies, in whom the detection of metastatic disease has a major impact on initial staging and assessment of response to therapy. The major sources of controversy regarding the use of CT for evaluation of possible metastases have been its limited specificity and cost-benefit ratio.

CT is clearly more sensitive than chest radiographs in diagnosing multiple pulmonary nodules in patients with suspected metastasis. The sensitivity of CT for detecting surgically proven nodules ranges from 50% to 75% (i.e., 50% to 75% of all resected nodules are seen on preoperative CT scans), whereas the sensitivity of chest radiographs is about 25%. In some studies, most nodules (55% to 60%) seen on CT and subsequently resected have proved to be benign (granulomas, intrapulmonary lymph nodes, and the like). In other studies, most CT-detected nodules (80% to 95%) have proved to be metastatic lesions. In an older study from the pre-MSCT era involving radiologic-surgical correlation, Peuchot and Libshitz evaluated 84 patients with previously documented extrathoracic malignancies and newly identified pulmonary nodules. These authors noted important limitations in both the sensitivity and specificity of CT. Of a total of 237 nodules resected, CT was able to identify only 173 (73%); 207 (87%) were metastatic tumors, 21 (9%) were benign, and 9 (4%) were bronchogenic carcinomas. Of 65 nodules interpreted as solitary on chest radiographs, CT disclosed multiple nodules in 46%, and 84% of these additional nodules were metastases. Although exquisitely sensitive for small nodule detection, CT is not infallible. In a study of 53 preoperative patients underoing 60 metastectomy surgeries from 1996-2004, in whom nodules detected at chest CT were compared with pathologically confirmed metastases, 46% to 47% of surgically palpable lung metastases were not reported at preoperative CT. Although there are intrinsic biases affecting such reports, including the possibility that surgical palpation will detect benign nodules and may overlook some malignant nodules that will subsequently manifest as growing nodules at chest CT, as well as the inherent difficulties correlating the location of nodules detected at chest CT with pathologic specimens, the results of this study nevertheless highlight the limitations of chest CT for small nodule detection and characterization.
Accordingly, CT results must be interpreted in light of the clinical characteristics of the patient. Primary tumors that commonly spread to the lungs, more advanced malignancies, and the absence of any simulators of metastases (silicosis, sarcoidosis, and prior granulomatous infection) would favor malignancy in pulmonary nodules detected by CT. Furthermore, smaller and less numerous nodules are more likely to be benign. The findings on CT are in themselves not specific, however, and follow-up may be necessary to demonstrate the growth or stability of small nodules.
Lung Cancer Staging
In patients with lung cancer, accurate anatomic staging is essential for planning the therapeutic approach. CT is used in both assessment of primary tumor extent and detection of lymph node metastases. However, its accuracy in both situations is limited. Lung cancer staging is reviewed in detail in Chapter 53 .
Computed Tomography
The primary goal of CT is to help distinguish patients who likely have resectable tumors from those who do not. Pulmonary malignancy is considered to be likely unresectable (stage T4) if the primary tumor (1) involves the trachea ( eFig. 18-31 ) or carina; (2) invades the mediastinum ( eFig. 18-32 ) with involvement of mediastinal structures ( eFig. 18-33 ); (3) invades the chest wall with involvement of great vessels, brachial plexus (see eFig. 53-18 ), or vertebral column ( eFig. 18-34 ); or (4) results in a malignant pleural effusion or pleural implants ( eFig. 18-35 , see eFigs. 53-4 and eFig. 53-5 ). Note, however, that acceptable morbidity and mortality have been reported following en bloc vertebral body resection with reconstruction for neoplasms invading the spine, particularly following induction chemotherapy, but such extensive surgeries are relatively restricted to larger academic or high-volume centers. The presence of satellite nodules in the same lobe as the primary tumor (see eFig. 53-19 ) is now designated as T3 in the seventh edition of the TNM Classification of Malignant Tumors and does not preclude surgical resection.
Tracheal (see eFig. 18-31 ) or carinal invasion ( eFig. 18-36 ) can be suggested with CT but requires biopsy confirmation. The CT diagnosis of chest wall (see eFig. 53-16 ) or mediastinal (see eFig. 18-32 ) invasion can be a problem, and many CT scans suggesting chest wall invasion are relatively nonspecific and can be seen with a number of inflammatory disorders. The sensitivity and specificity of CT for diagnosing T4 mediastinal or chest wall invasion are about 60% and 90%, respectively, although some findings (e.g., vertebral body destruction, encasement of mediastinal structures, mediastinal fat infiltration) are virtually diagnostic. Limited chest wall or mediastinal invasion is considered potentially resectable by many surgeons, but knowledge of tumor extent is nonetheless an important factor when planning therapy.
CT findings suggesting mediastinal invasion include (1) replacement of mediastinal fat by soft-tissue attenuation; (2) compression or displacement of mediastinal vessels by tumor; (3) tumor contacting more than 90 degrees of the circumference of a structure such as the aorta or pulmonary artery (the greater the extent of circumferential contact, i.e., 180 degrees, the greater the likelihood of invasion); (4) more than 3 cm of contact between tumor and the mediastinum; and (5) obliteration of the mediastinal fat plane normally seen adjacent to most mediastinal structures (see eFig. 18-32 and eFig. 18-37 ). However, individually, these findings are unreliable for differentiating mediastinal invasion from anatomic contiguity.
CT is of little value in the diagnosis of malignant pleural effusion because this diagnosis requires cytologic confirmation. However, in some patients, CT may show diffuse or nodular pleural thickening with (see eFig. 53-4 ) or without (see eFig. 18-35 and eFig. 53-5 ) pleural liquid. PET may show tracer uptake in patients with malignant pleural effusion (see eFig. 53-4 ), even when CT does not show evidence of nodular pleural thickening and when cytologic analysis is negative.
The diagnosis of mediastinal lymph node metastasis by CT is determined largely by node size, although the preservation of fat within lymph nodes often reflects the absence of pathologic infiltration, whereas necrosis may imply pathologic infiltration even within normal-sized lymph nodes. By convention, a nodal short axis diameter of 1 cm or greater is considered to be abnormal in all node stations ( Fig. 18-16 ), except the subcarinal space. In general a tumor cannot be detected in normal-sized lymph nodes by CT, and there are no characteristic appearances allowing benign and malignant causes of nodal enlargement to be distinguished (see eFigs. 53-1 and 53-3 ). Pooled information from 35 studies published between 1991 and June 2006 evaluating the performance of CT scanning for noninvasive staging of the mediastinum has shown that this size threshold has a sensitivity of only about 51% and a specificity of 86% for diagnosing mediastinal lymph node metastases in patients with lung cancer. Furthermore, the accuracy of CT for detecting involvement of individual node groups is low, perhaps as low as 40%. In a more recent review of the accuracy of mediastinal lymph node staging using CT, a combination of studies evaluating 7368 patients found the median sensitivity and specificity for mediastinal lymph node metastases detection at chest CT of 55% and 81%, respectively.
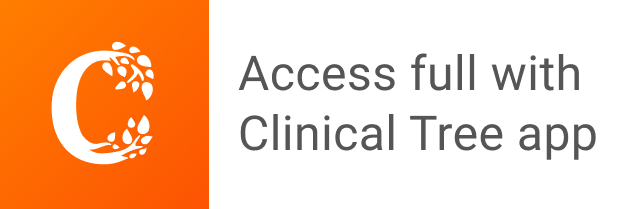