Abstract
Pulmonary surfactant is a complex substance with multiple functions in the microenvironments of the alveoli and small airways. The traditional functions of surfactant are biophysical activities to keep the lungs open, to decrease the work of breathing, and to prevent alveolar edema. Most of the components of surfactant also contribute to innate host defenses and to injury responses of the lung. Surfactant deficiency states occur with prematurity and with severe lung injury syndromes. Recent studies in humans and in mice are defining an expanding number of genetic and metabolic abnormalities that disrupt the surfactant and cause lung diseases, which range from lethal respiratory failure at birth to chronic interstitial lung disease in later life. This chapter summarizes the aspects of surfactant biology that are relevant to children.
Keywords
pulmonary surfactant, SP-A, SP-B, SP-C, SP-D, acute respiratory distress syndrome, respiratory distress syndrome, bronchopulmonary dysplasia
Pulmonary surfactant is a complex substance with multiple functions in the microenvironments of the alveoli and small airways. The traditional functions of surfactant are the biophysical activities to keep the lungs open, to decrease the work of breathing, and to prevent alveolar edema. Most of the components of surfactant also contribute to innate host defenses and injury responses of the lung. Surfactant deficiency states occur with prematurity and with severe lung injury syndromes. Recent studies in humans and in mice have defined an expanding number of genetic and metabolic abnormalities that disrupt surfactant and cause lung diseases, which range from lethal respiratory failure at birth to chronic interstitial lung disease in later life. We will summarize those aspects of surfactant biology that are relevant to children.
Surfactant Composition and Metabolism
Composition
Surfactant recovered from lungs by bronchoalveolar lavage contains about 80% phospholipids, about 8% protein, and about 8% neutral lipids, primarily cholesterol ( Fig. 5.1 ). The phosphatidylcholine species of the phospholipids contribute about 70% by weight to surfactant. The phospholipids in surfactant are unique relative to the lipid composition of lung tissue or other organs. About 50% of the phosphatidylcholine species have two palmitic acids or other saturated fatty acids esterified to the glycerol-phosphorylcholine backbone, resulting in “saturated” phosphatidylcholine, which is the principal surface-active component of surfactant. About 8% of surfactant is the acidic phospholipid phosphatidylglycerol. Surfactant from the immature fetus contains relatively large amounts of phosphatidylinositol, which then decreases as phosphatidylglycerol appears with lung maturity.

Four primary surfactant-associated proteins have been identified: A, B, C, and D. Initial analyses of these proteins suggested that the hydrophilic surfactant protein A (SP-A) and surfactant protein D (SP-D) were primarily involved in pulmonary innate immunity, whereas the hydrophobic surfactant protein B (SP-B) and surfactant protein C (SP-C) facilitated surfactant lipid physiology. However, we now know that the surfactant proteins often cross these lines of functional classification.
SP-A and SP-D are members of the collectin family of innate defense proteins. Collectins are defined by four structural domains shared by all family members: a short amino-terminal cross-linking domain, a triple helical collagenous domain, a neck domain, and a carbohydrate recognition domain (CRD). Three neck domains combine and facilitate the formation of a collagen-like triple helix that then aggregates to form larger multimers of the collectin trimer. SP-A is a 24-kD monomer that further assembles to a bouquet of six trimers with a molecular size of 650 kD. SP-A is encoded by two genes located within a “collectin locus” on the long arm of chromosome 10 that also includes the genes for SP-D and the mannose binding protein. In humans, SP-A synthesis begins during the second trimester of gestation and occurs primarily in the alveolar type II epithelial cells, club cells, and in cells of tracheo-bronchial glands. SP-A is required for the formation of tubular myelin and has several roles in pulmonary host defense.
SP-D is a 43-kD hydrophilic collectin with a monomer structure that is similar to SP-A, although the collagen domain of SP-D is much longer. Structural studies demonstrate that SP-D trimers further combine into larger multimeric complexes through N-terminal interactions that are stabilized by N-terminal disulfide bonds. Although larger, more complex forms have been identified, SP-D exists predominately as a tetramer of trimeric subunits (dodecamer) assembled into a cruciform. SP-D is synthesized by type II cells and by club cells, as well as in other epithelial sites. Like the other surfactant proteins, SP-D expression is developmentally regulated and induced by glucocorticoids and inflammation. In addition to the complex roles of SP-D in pulmonary host defense, SP-D also influences surfactant structure and is required for surfactant reuptake and the regulation of pulmonary surfactant pool sizes.
SP-B is a small hydrophobic protein that contributes about 2% to the surfactant mass. The SP-B gene is on human chromosome 2 and is expressed in a highly cell-specific manner. The primary translation product is 40 kD, but the protein is clipped in the type II cell to become an 8-kD protein prior to associating with phospholipids during the formation of lamellar bodies. SP-B facilitates the surface absorption of lipids into the expanding alveolar surface film and enhances their stability during the movements of the respiratory cycle. A genetic lack of SP-B causes a loss of normal lamellar bodies in type II cells, a lack of mature SP-C, and the appearance of incompletely processed SP-C in the airspaces.
The SP-C gene is on chromosome 8, and its primary translation product is a 22-kD protein that is processed to an extremely hydrophobic 35 amino acid peptide rich in valine, leucine, and isoleucine. The SP-C gene is expressed in cells lining the developing airways from early gestation. With advancing lung maturation, SP-C gene expression becomes localized only to type II cells. SP-B and SP-C are packaged together into lamellar bodies and function cooperatively to optimize rapid adsorption and spreading of phospholipids. Surfactants prepared by organic solvent extraction of natural surfactants or from lung tissue contain SP-B and SP-C. Such surfactants are similar to natural surfactants when evaluated for in vitro surface properties or for function in vivo.
Surfactant Metabolism and Secretion
The synthesis and secretion of surfactant by the type II cell is a complex sequence that results in the release by exocytosis of lamellar bodies to the alveolus. Enzymes within the endoplasmic reticulum (ER) use glucose, phosphate, and fatty acids as substrates for phospholipid synthesis. The details of how the surfactant lipids condense with SP-B and SP-C to form the surfactant lipoprotein complex within lamellar bodies remain obscure. Ultrastructural abnormalities of type II cells with SP-B deficiency and ABCA3 deficiency in full-term infants indicate that these gene products are essential for lamellar body formation. A basal rate of surfactant secretion occurs continuously, and surfactant secretion can be stimulated by β-agonists and purines, or with lung distention and hyperventilation.
The alveolar pool size of surfactant is about 4 mg/kg in the adult human. The lung tissue of the adult human contains much more surfactant, and only about 7% of the surfactant lipids are in the secreted pool. The surfactant pool size per kilogram probably changes little with age after the newborn period. While no estimates exist for the full-term human, full-term animals have alveolar pool sizes of about 100 mg/kg, and this large pool decreases to adult values by about 1 week of age. The alveolar surfactant pool size in the adult (and presumably young child) is small relative to other mammalian species (e.g., about 30 mg/kg in adult sheep), which may make the human lung more susceptible to surfactant deficiency with lung injury. Infants with respiratory distress syndrome (RDS) have alveolar surfactant pool sizes of less than 5 mg/kg.
The kinetics of surfactant metabolism have been extensively studied in adult, term, and preterm animal models. In all species studied to date, including primates, the surfactant component synthesis to secretion interval is relatively long, and the alveolar half-life of newly secreted surfactant is very long, on the order of 6 days in healthy newborn lambs. The surfactant components are recycled back into type II cells, and recycling is more efficient in newborn than adult animals. These observations have been validated by quite extensive studies in preterm and term humans using stable isotopes to label surfactant precursors or components. A limitation of the studies is the need to have an endotracheal tube in place to allow repetitive sampling of lung fluid. Depending on the labeled precursor, the time from synthesis to peak secretion of new surfactant ranged from 2 to 3 days, and the half-life for clearance was 2 to 4 days in preterm infants. Similar values were measured for term infants. In general, preterm or term infants with lung disease have surfactant with smaller pool sizes, less synthesis and secretion, and shorter half-life values. These measurements include term infants with pneumonia, meconium aspiration syndrome, and congenital diaphragmatic hernia. There are no measurements of surfactant metabolism for older children. In one report in normal adults using sputum samples, peak labeling of surfactant phosphatidylcholine occurred about 2 days after the labeled precursor was given, and the subsequent half-life was about 7 days. These studies demonstrate that the replacement of endogenous surfactant pools is slow, and alveolar pools turn over slowly.
Alveolar Life Cycle of Surfactant
After secretion, surfactant goes through a series of form transitions in the airspace ( Fig. 5.2 ). The lamellar bodies unravel to form the elegant structure called tubular myelin. This lipoprotein array has SP-A at the corners of the lattice and requires at least SP-A, SP-B, and the phospholipids for its unique structure. Tubular myelin and other large surfactant lipoprotein structures are the reservoir in the fluid hypophase for the formation of the surface film within the alveolus and small airways. The hypophase is a very thin fluid layer covering the distal epithelium with a volume of about 0.5 mL/kg body weight that has a surfactant concentration of approximately 10 mg/mL. New surfactant enters the surface film, and “used” surfactant leaves in the form of small vesicles. The surface-active tubular myelin contains SP-A, SP-B, and SP-C, while the biophysically inactive small vesicles that are recycled and catabolized contain very little surfactant protein. The total surfactant pool size is less than the amount of active surfactant because 30% to 50% of the alveolar phospholipids are in catabolic forms in the normal lung. Pulmonary edema and products of lung injury can accelerate form conversion and cause a depletion of the surface-active fraction of surfactant despite normal or high total surfactant pool sizes. Surfactant is catabolized primarily by type II cells and alveolar macrophages. Granulocyte-macrophage colony-stimulating factor deficiency prevents alveolar macrophages from catabolizing surfactant and results in the clinical syndrome of alveolar proteinosis. The important concept is that the alveolar pool of functional surfactant is maintained by dynamic metabolic processes that include secretion, reuptake, and resecretion balanced by catabolism.

Surfactant Function
Alveolar Stability
Alveoli are polygonal with flat surfaces and curvatures where the walls of adjacent alveoli intersect. Alveoli are interdependent in that their structure is determined by the shape and elasticity of neighboring alveolar walls. The forces acting on the pulmonary microstructure are chest wall elasticity, lung tissue elasticity, and surface tensions of the air-fluid interfaces of the small airways and alveoli. Although the surface tension of surfactant decreases with surface area compression and increases with surface area expansion, the surface area of an alveolus changes little with tidal breathing. The low surface tensions resulting from surfactant help to prevent alveolar collapse and keep interstitial fluid from flooding the alveoli. Surfactant also keeps small airways from filling with fluid and thus prevents the potentially ensuing luminal obstruction. If alveoli collapse or fill with fluid, the shape of adjacent alveoli will change, which can result in distortion, overdistention, or collapse. When positive pressure is applied to a surfactant-deficient lung, the more normal alveoli will tend to overexpand, and the alveoli with inadequate surfactant will collapse, generating a nonhomogeneous inflated lung.
Pressure-Volume Curves
The static effects of surfactant on a surfactant-deficient lung are evident from the pressure-volume curve of the preterm lung ( Fig. 5.3 ). Preterm surfactant-deficient rabbit lungs do not begin to inflate until pressures exceed 20 cm H 2 O. The pressure needed to open a lung unit is related to the radius of curvature and surface tension of the meniscus of fluid in the airspace leading to the lung unit. The units with larger radii and lower surface tensions will “pop” open first because, with partial expansion, the radius increases and the forces needed to finish opening the unit decrease. Surfactant decreases the opening pressure from greater than 20 to 15 cm H 2 O in this example with preterm rabbit lungs. Because surfactant does not alter airway diameter, the decreased opening pressure results from surface adsorption of the surfactant to the fluid in the airways. The inflation is more uniform as more units open at lower pressures, resulting in less over-distention of the open units.

A particularly important effect of surfactant on the surfactant-deficient lung is the increase in maximal volume at maximal pressure. In this example, maximal volume at 30 cm H 2 O is increased over two times with surfactant treatment. Surfactant also stabilizes the lung on deflation. The surfactant-deficient lung collapses at low transpulmonary pressures, whereas the surfactant-treated lung retains about 30% of the lung volume on deflation. This retained volume is similar to the total volume of the surfactant-deficient lung at 30 cm H 2 O and demonstrates how surfactant treatments increase the functional residual capacity (FRC) of the lung.
Host Defense Functions of Surfactant
SP-A and SP-D are pattern recognition molecules that bind a variety of polysaccharides, phospholipids, and glycolipids on the surface of bacterial, viral, and fungal pathogens. SP-A and SP-D binding forms protein bridges between microbes that induce microbial aggregation and stimulate the recognition, uptake, and clearance of pathogens by host defense cells. In addition, SP-A and SP-D binding may have direct antibacterial and antifungal activity by increasing pathogen membrane permeability.
Although binding and aggregation of infectious microbes is a critical feature of SP-A and SP-D physiology, these proteins also have more complex roles in host defense. SP-A and SP-D have been implicated in the stimulation and inhibition of several immune pathways. Both SP-A and SP-D bind CD14 and inhibit lipopolysaccharide-induced expression of proinflammatory cytokines through CD14 and toll-like receptor 4. SP-A binds toll-like receptor 2 and inhibits proinflammatory cytokine release in response to peptidoglycan. Gardai and colleagues proposed a model by which SP-A and SP-D might stimulate or inhibit inflammation through the competing actions of signal regulating protein α (SIRPα) and calreticulin/CD91. Their model suggests that in the unbound state, the CRDs of SP-A or SP-D inhibit macrophage activation by binding to SIRPα, which inhibits activation of nuclear factor κB (NFκB). In contrast, if the CRDs of SP-A or SP-D are occupied by a microbial ligand, binding to SIRPα is inhibited and instead the collectins bind to the macrophage activating receptor, calreticulin/CD91, which turns on NFκB and subsequently induces proinflammatory mediator release and alveolar macrophage activation. SP-A also may contribute to adaptive immune responses. SP-A inhibits the maturation of dendritic cells in response to potent T cell stimulators and enhances the endocytic ability of dendritic cells. In addition, SP-A downregulates lymphocyte activity and proliferation.
The hydrophobic surfactant proteins SP-B and SP-C may also have host defense functions. Although SP-B can inhibit bacterial growth in vitro, overexpression of SP-B or reduced expression of SP-B in the lungs of mice did not alter bacterial clearance, suggesting that SP-B is not involved in innate host defense. However, elevated levels of SP-B in the lungs of endotoxin-exposed mice decreased pulmonary inflammation. Thus, SP-B may contribute to the modulation of inflammation in the injured lung. SP-C binds lipopolysaccharide and blocks the production of tumor necrosis factor-α by macrophages. However, possible roles for SP-C in bacterial clearance or lung inflammation in vivo have not been evaluated.
Surfactant Deficiency
The Preterm Infant With Respiratory Distress Syndrome
RDS in preterm infants is a primary surfactant deficiency that initially does not include lung injury, unless antenatal infection complicates the lung disease. The surfactant system normally is mature by about 35 weeks’ gestation, but the early appearance of surfactant and lung maturation is frequent in infants delivered prematurely. Early maturation is thought to occur in response to fetal stress resulting in increased fetal cortisol levels, or by exposure of the fetal lung to inflammation as a result of chorioamnionitis. Maternal treatments with corticosteroids are routinely given to decrease the risk of RDS if preterm delivery before 32 to 34 weeks’ gestation is anticipated. Induced lung maturation includes not only an induction of surfactant but also thinning of the mesenchyme, which increases lung gas volumes. Unless preterm infants have early lung maturation, they develop progressive respiratory distress from birth, which is characterized by tachypnea, grunting, increased work of breathing, and cyanosis. Infants who have died of RDS have alveolar pool sizes of surfactant of less than 5 mg/kg. Although this amount is similar to the amount of surfactant recovered from healthy adult humans, surfactant from the preterm infant has decreased function, probably because it contains less of the surfactant proteins, which are critical for biophysical function. The surfactant from the preterm infant also is more susceptible to inactivation by edema fluid, and the preterm lung is easily injured if a stable FRC is not maintained or if the lung is overstretched.
The Injured Mature Lung
Acute respiratory distress syndrome (ARDS) describes an overwhelming inflammatory reaction within the pulmonary parenchyma leading to global lung dysfunction. ARDS is defined by acute onset, an oxygenation index less than 200, bilateral infiltrates on chest x-ray, and a pulmonary capillary wedge pressure of less than 18 mm Hg, or the absence of clinical evidence for left-sided heart failure. The etiology of ARDS is multifactorial and can occur in association with lung injury secondary to trauma, sepsis, aspiration, pneumonia, massive blood transfusions, or near drowning to name some associations. It is a common disease, affecting approximately 15% to 20% of all patients ventilated in the adult intensive care unit (ICU) and 1% to 4.5% of patients in the pediatric ICU. ARDS has a high mortality rate of 40% to 50%.
Impairment of surfactant with ARDS can result from inhibition, degradation, or decreased production. The proteinaceous pulmonary edema characteristic of ARDS can inactivate surfactant by dilution and by competition for the interface. Plasma proteins known to inhibit surfactant function include serum albumin, globulin, fibrinogen, and C-reactive protein. In addition to proteins, phospholipases, along with their products, fatty acids, and lipids inhibit surface activity. Epithelial cell injury by inflammatory mediators can decrease surfactant production and contribute to surfactant deficiency. Normally in the lung, about 50% of surfactant is present in the bioactive form that has a high SP-B and SP-C content. In ARDS, small vesicular forms increase, and the pool of active surfactant is depleted.
The phospholipid content is decreased and the phospholipid composition is abnormal in bronchoalveolar lavage fluid (BALF) from patients with ARDS. SP-A, SP-B, and SP-C also are decreased in BALF from patients with ARDS. The surfactant protein levels can remain low for at least 14 days after the onset of ARDS. Changes in surfactant composition, including phospholipids, fatty acids, and proteins, likely represent alveolar type II cell injury with altered metabolism, secretion, or recycling of components. SP-A and SP-B concentrations are also reduced in the lungs of patients at risk for ARDS, even before the onset of clinical lung injury. In contrast, SP-D levels in BALF remain normal, except in a subgroup of patients who later died. Decreased SP-D levels in BALF were 85.7% sensitive and 74% specific in predicting death from ARDS.
Genetic Deficiencies of Surfactant in Mice and Humans
Mice with targeted deletion of the Sftpa gene (Sftpa −/− ) for SP-A protein survived normally without changes in surfactant composition, function, secretion, and reuptake; however, there was no tubular myelin. Although seemingly normal at baseline, significant defects were detected in pulmonary host defense in SP-A-deficient mice when they were subjected to a microbial challenge. The clearance of group B Streptococcus, Haemophilus influenzae, respiratory syncytial virus (RSV), and Pseudomonas aeruginosa was delayed in Sftpa −/− mice, and the recognition and uptake of bacteria by alveolar macrophages were deficient. Oxygen radical production and killing of engulfed microorganisms by Sftpa −/− macrophages were markedly reduced, while markers of lung inflammation were increased following infection in Sftpa −/− mice.
Despite the considerable innate immune defects that are associated with SP-A deficiency in animal models, we have yet to find a human susceptibility to pulmonary infection that is caused by a Sftpa mutation. However, polymorphisms in the human genes for SP-A affecting their function have been identified, and humans with these polymorphisms have increased susceptibility to acquiring infections with RSV and Mycobacterium tuberculosis. Analyses suggest that SP-A polymorphisms may also affect infection severity since patients that are homozygous or heterozygous for asparagine at amino acid position 9 are more likely to need intensive care, mechanical ventilation, or longer hospitalization. Although there are no clear associations between Sftpa mutation and pulmonary infection, an association has been reported between a rare heterozygous mutation in the Sftpa and adult onset interstitial pulmonary fibrosis and lung cancer. It is likely that these mutations cause SP-A misfolding and the trapping of SP-A in the ER, which leads to chronic ER stress and cell injury.
Mice with deletion of the Sftpd gene (Sftpd −/− ) for SP-D protein survived normally, but unlike SP-A-deficient mice that had relatively normal lungs at baseline, Sftpd −/− mice spontaneously developed pulmonary inflammation and airspace enlargement. In addition, Sftpd −/− mice accumulated increased numbers of apoptotic macrophages, and enlarged, foamy macrophages that released reactive oxygen species and metalloproteinases. When Sftpd −/− mice were exposed to a microbial challenge, the uptake and clearance of viral pathogens including influenza A and RSV were deficient, whereas the clearance of group B Streptococcus and H. influenzae were unchanged. However, oxygen radical release and production of the proinflammatory mediators were increased in Sftpd −/− mice when exposed to either viral or bacterial pathogens indicating that SP-D plays an antiinflammatory role in the lung that is independent of the clearance of pathogens. SP-D deficiency has not been described in humans, but polymorphisms at amino acid position 11 are associated with increased risk of RSV infection.
Gene-targeted mice lacking SP-B, and infants with hereditary SP-B deficiency, demonstrate the critical role of SP-B in surfactant function, homeostasis, and lung function. Targeted disruption of the mouse SP-B gene causes respiratory failure at birth. Despite normal lung structure, the SP-B-deficient mice failed to inflate their lungs postnatally. Type II cells of SP-B-deficient mice had large multivesicular bodies but did not have lamellar bodies, and the proteolytic processing of pro-SP-C (the preprocessed form of SP-C) was disrupted. Infants with SP-B deficiency die from respiratory distress in the early neonatal period with the same anatomic findings. Mutations leading to partial SP-B function have been associated with chronic lung disease in infants. Because SP-B is required for both intracellular and extracellular aspects of surfactant homeostasis, SP-B deficiency has not been treated successfully with surfactant replacement therapy, and survival is dependent on lung transplantation. It is important to note that mice and infants without the adenosine triphosphate-binding cassette transporter A3 (ABCA3) have type II cells without lamellar bodies and the same lethal respiratory failure phenotype as observed in SP-B deficiency.
SP-C-deficient mice survive and have normal surfactant composition and amounts. However, surfactant isolated from the SP-C-deficient mice forms less stable bubbles, demonstrating a role for SP-C in developing and maintaining lipid films. SP-C mutations were recently identified in patients with familial and sporadic interstitial lung disease. Sftpc mutations in these patients alter the ability of the protein to fold correctly and result in the retention of SP-C in the ER and the subsequent development of ER stress which, in turn, leads to pulmonary cell injury and death. Histological features of lung disease in these individuals include lungs with a thickened interstitium, infiltration with inflammatory cells and macrophages, fibrosis, and abnormalities of the respiratory epithelium. In isolated reports, infants with respiratory failure from SP-D deficiency have been supported by tracheostomy and chronic mechanical ventilation for years prior to eventual decannulation and survival off ventilator support.
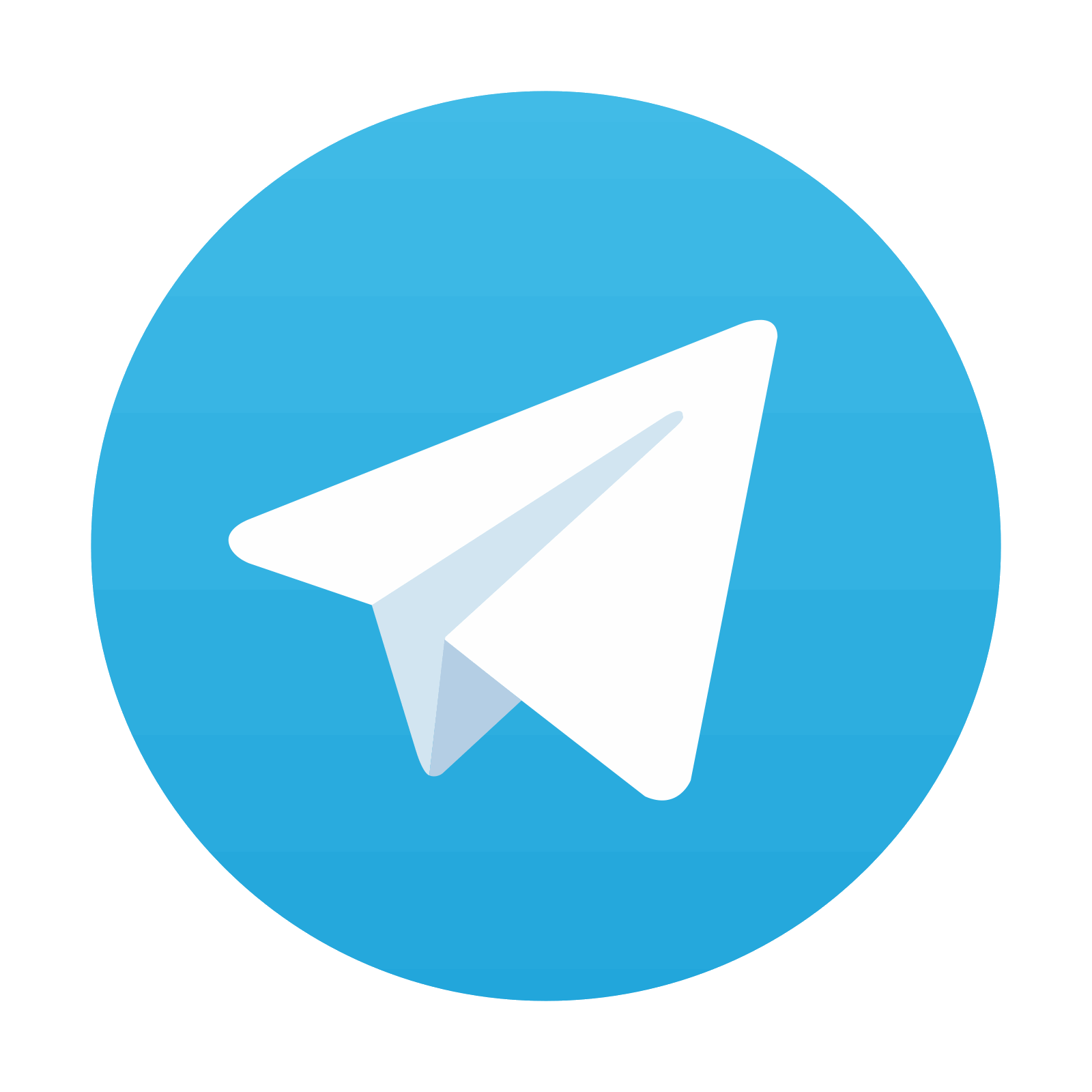
Stay updated, free articles. Join our Telegram channel
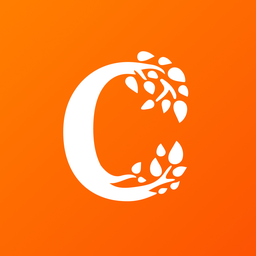
Full access? Get Clinical Tree
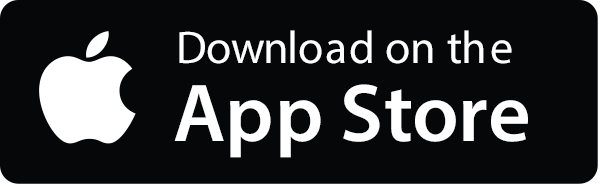
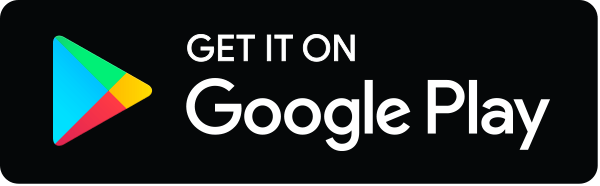