Abstract
Disorders of the Respiratory Tract in Children outlines the congenital abnormalities and diseases that affect the respiratory health of children. A thorough understanding of the structure, function, and development of the normal lung is essential for both understanding the pathophysiologic mechanisms underpinning these disorders and how they result in the signs and symptoms of, and the approach to therapy for, respiratory diseases. This chapter outlines the gross and microscopic anatomy of the normal lung and lung physiology. Cellular and molecular biology will be described in other chapters.
Keywords
anatomy of the lungs, pulmonary circulation, airways, innervation of the lungs, growth and development of the lungs, ventilation, perfusion, properties of gases, elastic recoil, lung compliance, airway resistance, gas exchange, dead space, diffusion, systemic gas transport, oxygen therapy, tissue respiration, metabolic functions of the lung
Knowledge of the normal development, structure, and physiologic function of the lungs is required to understand the pathophysiology that is seen in disease. Historically, the understanding of lung function was derived solely from clinical observation and postmortem histologic examination. The development of invasive and noninvasive techniques that were capable of assessing lung structure and function in living subjects greatly improved our understanding of lung physiology on an “organ basis.” There has been an explosion of knowledge in cellular and molecular biology, which is covered in detail in other chapters. This chapter will focus on the normal structure of the lung and organ physiology.
Normal Lung Anatomy and Cell Function
Knowledge of normal lung anatomy is one of the basic requirements for understanding lung function in health and disease. Because detailed descriptions of lung anatomy are available elsewhere, this section will focus on selected aspects of gross and microscopic anatomy to enable the reader to understand the physiologic changes that occur in congenital and acquired lung disease.
The shape of the lung reveals three faces: the convex costal face opposed to the rib cage, the concave diaphragmatic face resting on the diaphragmatic dome, and the mediastinal face, where the right and left lung are oriented toward each other. The right and left lung are each embedded in a separate pleural cavity and are separated by the mediastinum. Except at the hilum (where airways, vessels, and nerves enter or leave the lung), the lung’s outer surface is covered by the visceral pleura, which also extends into the fissures, thereby demarcating the pulmonary lobes ( Fig. 6.1 ).

Airways
The airways are composed of two functional compartments. A proximal conducting zone (the bronchial tree) continuously connects to a distal respiratory zone (the alveolar region), where gas exchange takes place. The basic structure of the airways is already present at birth; therefore, neonates and adults share a common bronchopulmonary anatomy ( Figs. 6.2 and 6.3 ). When airways divide, they do so by dichotomous branching, over an average of 23 generations, although the number of times that branching occurs varies. This airway variability has physiologic implications; different pathways will have different resistances to air flow, and a heterogeneous distribution of gases or inhaled particles may occur. As the bronchi branch and decrease in size, they lose their cartilage and become bronchioles. Ultimately, a terminal bronchiole opens into the alveoli-containing gas-exchanging area of the lung. In the human, the gas-exchanging area begins with several generations of respiratory bronchioles (i.e., bronchioles with alveoli attached to their wall) that connect to alveolar ducts whose “wall” completely consists of alveolar openings. The most distal alveolar ducts end in blind alveolar sacs. The unit of lung parenchyma distal to a terminal bronchiole (i.e., the unit in which all airways participate in gas exchange) is termed the acinus ( Fig. 6.4 ).



The airways are lined with a continuous epithelium that gradually changes from a ciliated pseudostratified columnar epithelium in the bronchi to a ciliated simple cuboidal epithelium in smaller bronchioles near the gas-exchanging units. At the transition into the alveolar region, the epithelium abruptly becomes squamous. At all levels, the epithelium is not made of a single cell type, but rather a mosaic of several cell types: lining cells and secretory cells, often with rarer cells with specialized functions interspersed ( Fig. 6.5 ). Ciliated cells predominate throughout the bronchial and bronchiolar epithelium and are responsible for propelling mucus from the peripheral airways to the pharynx ( Fig. 6.6 ). This mucociliary transport system is an important defense mechanism of the lungs. The mucous layer has two parts, a superficial gel layer with high viscosity and a deeper periciliary layer with a brushlike structure. The cilia form a dense, long carpet on top of the epithelial cells, and their coordinated to-and-fro action propels the gel mucous layer toward the oropharynx. Cilia are a derivative of the centrioles, and there are approximately 200 of them on the apex of each ciliated cell. The cilia are anchored within the cell with a basal body that is oriented in the direction of mucous movement. The shaft of the cilium has a central pair of single tubules that are connected via radial spokes to nine peripheral pairs of tubules. The tip of the cilium has tiny hooklets that probably help grab the gel component of the mucous layer and propel it forward. The cilium has a beat frequency of 12 to 20 Hz and is coordinated both with other cilia on that cell and concurrently with the cilia on adjacent cells to yield a synchronized wave flowing up the airway. Primary ciliary dyskinesia (PCD) is a group of disorders that includes Kartagener syndrome and the erroneously named immotile cilia syndrome. In PCD, there are defects within the tubules, in their inner or outer dynein arms, or in the radial arms that result in a disorganized movement of the cilia that precludes normal mucociliary transport and results in chronic bronchitis and repeated pneumonias (see Chapter 71 ). Submucosal glands, which are present in large and small bronchi, are the chief source of airway secretions and contain both serous and mucus cells. Goblet cells are seen in the trachea and bronchi (see Fig. 6.6 ). They produce mucin, a viscous mixture of acid glycoproteins that contributes to the mucous layer. Submucosal glands and goblet cells can increase in number in disorders such as chronic bronchitis, the result being mucous hypersecretion and increased sputum production. The basal cell, commonly seen within the pseudostratified columnar bronchial epithelium resting on the basement membrane but not reaching the lumen, is undifferentiated and acts as a precursor of ciliated or secretory cells (see Fig. 6.6 ).


Whereas ciliated cells are still present in smaller bronchioles, goblet cells are gradually replaced by nonciliated bronchiolar epithelial cells (often termed club cells). These cells are characterized by their dome-shaped apex that protrudes into the airway lumen and by secretory granules. Their secretory products, which include the club cell secretory protein (CCSP), add to the bronchiolar lining layer. CCSP is thought to have immunomodulatory functions. In addition, club cells are a site of cytochrome P-450–dependent detoxification of xenobiotics, and they act as progenitor cells for the maintenance of the bronchiolar epithelium.
There are several rarer cell types found within the airways; however, their functional significance is less well understood. The brush cell, which is only rarely found in the human lung, has a dense tuft of broad, short microvilli. It is supposed to “taste” the chemical composition of the airway lining fluid. Neuroendocrine cells secrete mediators into subepithelial capillaries. Sometimes these cells are organized in clusters (neuroepithelial bodies) that are thought to have oxygen-sensing functions. Neuroepithelial bodies are found more frequently within the fetal airways or in pediatric disorders characterized by chronic hypoxemia (e.g., bronchopulmonary dysplasia).
Histologically, the remainder of the airway consists of the submucosa, with its network of blood vessels and nerves, and a variable amount of smooth muscle and cartilage. Within the submucosa are mast cells containing vasoactive peptides and amines, cells of the immune system (plasma cells, lymphocytes, and phagocytes), and submucosal glands. In the main stem bronchi, cartilage is present in C -shaped rings. However, as further branching of bronchi occurs, progressively less cartilage is present as plates. Cartilage adds structural rigidity to the airway and thus plays an important role in maintaining airway patency, especially during expiration. Congenital deficiency of airway cartilage and hence airway instability has been associated with bronchiectasis (Williams-Campbell syndrome) and congenital lobar hyperinflation (previously referred to as congenital lobar emphysema ).
The smooth muscle content of the airway also varies with its anatomic location. In the largest airways, a muscle bundle connects the two ends of the C -shaped cartilage. As the amount of cartilage decreases, the smooth muscle assumes a helical orientation and gradually becomes thinner, ultimately reaching the alveolar ducts where the smooth muscle cells lie in the alveolar entrance rings. Muscle contraction increases airway rigidity in all airways.
Although it has been widely assumed that the airway muscles of newborn infants are inadequate for bronchoconstriction, this assumption is not correct. Even premature infants have smooth muscle, and although the amount may be statistically less than that seen in adults, it is likely enough to constrict the infant’s much more compliant airways. Indeed, pulmonary function test results have demonstrated that airway resistance can be altered with bronchodilating drugs. The belief that infants have little or no smooth muscle in their airways is even less tenable in such disorders as Northway’s old bronchopulmonary dysplasia and left-to-right congenital heart disease, in which hypertrophy of the airway smooth muscle has been demonstrated by morphometric measurement. Congenital deficiency of large-airway smooth muscle and elastic fibers is associated with marked dilation of the trachea and bronchi, which promotes retention of airway secretions and ultimately leads to recurrent pulmonary sepsis, a condition known as the Mounier-Kuhn syndrome or tracheobronchomegaly.
Alveolar Region
Within the pulmonary lobule, defined as the smallest unit of lung structure marginated by connective tissue septae, one finds the lungs’ region for gas exchange. The terminal respiratory (gas-exchanging) unit consists of the structures distal to the terminal bronchiole: the respiratory bronchioles (bronchioles with alveoli budding from their walls), alveolar ducts, and alveoli ( Fig. 6.7 ).

As mentioned earlier in the chapter, the acinus is the portion of lung parenchyma distal to a terminal bronchiole (see Fig. 6.4 ). It is the basic functional gas-exchanging unit of the lung. The acinus is approximately 6 to 10 mm in diameter in the adult lung. In the adult lung, these units have a total gas volume of 2000 to 4000 mL and a surface area of about 140 m 2 ( Table 6.1 ), yet all alveoli are within 5 mm of the closest terminal bronchiole. True alveoli are not spherical but more closely resemble hexagons with flat, sheetlike surfaces. The average alveolar diameter ranges from 200 to 300 µm. Within the acini, interalveolar holes (termed pores of Kohn ) are present in alveolar walls. Although they have been thought to provide channels for collateral ventilation, the fact that pores of Kohn are covered with surfactant needs to be taken into consideration. Ultrastructural evidence suggests that they are used by alveolar macrophages to reach neighboring alveoli. In the newborn lung, there are few, if any, pores of Kohn. This might contribute to the fact that relative to adult lung, infant lung is more predisposed to patchy atelectasis.
Alveolar number | 480 million |
Alveolar surface area | 140 m 2 |
Capillary volume | 210 mL |
Air-blood barrier thickness | 2 µm |
The alveoli are lined by two types of epithelial cells ( Fig. 6.8 ). Type I alveolar epithelial cells are extremely broad, thin (0.1 to 0.5 µm) cells that in total cover about 95% of the alveolar surface. They are markedly differentiated cells that possess few organelles, and because their cytoplasmic leaflets are so thin, they provide an epithelial barrier for gas exchange that cannot be resolved by routine histology. Recent work has demonstrated that these cells are capable of actively transporting Na + with Cl − and water following, and thus participate in the clearance of airspace fluid (see Chapter 36 ). Type II epithelial cells are more numerous than type I cells, but because of their cuboidal shape, they occupy only about 5% of the total alveolar surface area ( Table 6.2 ). They are characterized histologically by microvilli and osmophilic inclusions termed lamellar bodies, which are storage sites for surfactant components (see Fig. 6.8 ).

Cell Type | Total Cells (%) | Cell Volume (µm 3 ) | Apical Surface Area (µm 2 ) |
---|---|---|---|
Epithelium Alveolar type I Alveolar type II | 8 16 | 1764 889 | 5098 183 |
Endothelium | 30 | 632 | 1353 |
Interstitial | 36 | 637 | |
Alveolar macrophages | 10 | 2492 |
The type II alveolar epithelial cell maintains homeostasis within the alveolar space in several ways. First, it is the source of pulmonary surfactant and as such is one index of fetal lung maturity; surfactant decreases the surface tension at the alveolar air-liquid interface. Second, in the postnatal lung, this cell is the precursor of the type I alveolar epithelial cell and thus plays a key role in the normal maintenance of the alveolar epithelium as well as in the repair process following lung injury when progenitor cells from type II alveolar epithelium contribute to alveolar renewal, repair and cancer. Third, it is capable of actively transporting ions against an electrochemical gradient and is involved in both fetal lung liquid secretion, in airspace fluid reabsorption at birth, and in the postnatal reabsorption of fluid from the airspace following the development of alveolar pulmonary edema (see the discussion on fetal lung liquid secretion in “ The Lung at Birth ” later in this chapter). Two pediatric disorders associated with the type II alveolar epithelial cell are (1) its lack of maturity and surfactant secretion in respiratory distress syndrome (RDS) of preterm infants and (2) its decreased lamellar body formation and surfactant secretion in surfactant dysfunction mutations (e.g., in genes encoding surfactant protein B or the lipid transporter ABCA3).
The cell junctions (zonulae occludentes) between type I and type II alveolar epithelial cells are very tight and thus restrict the movement of both macromolecules and small ions (e.g., sodium and chloride) (see Fig. 6.8 ). This tightness is an essential characteristic of the cells lining the alveolar space; it enables the active transport of ions. Also, these tight junctions provide a margin of safety for patients who are susceptible to pulmonary edema; significant interstitial pulmonary edema can be present without alveolar flooding, thus preserving gas exchange.
There is a thick side and a thin side to the alveolar air-blood barrier. Gas exchange is thought to occur predominantly on the thin side where there are only the thin extensions of type I alveolar epithelial cells, fused basement membranes, and capillary endothelial cells (see Fig. 6.8 ). Capillaries undergo considerable stress (e.g., during exercise or lung hyperinflation) and must have great tensile strength. This strength is mainly imparted by type IV collagen located in the basement membrane. The thick side of the barrier consists of connective tissue, amorphous ground substance, and scattered fibroblasts. The thick side, in addition to providing structural support, acts as a site of fluid and solute exchange.
Pulmonary Vascular System
The lung receives blood from both ventricles. In the postnatal lung, the entire right ventricular output enters the lung via the pulmonary arteries, and blood ultimately reaches the gas-exchanging units by one of the pulmonary arterial branching systems. Arterial branches accompany the bronchial tree and divide with it, each branch accompanying the appropriate bronchial division (see Fig. 6.3B ). In addition, supernumerary arteries take off at right angles and directly supply the gas-exchanging units (see Fig. 6.3C ). The pulmonary capillary bed is the largest vascular bed in the body and covers a surface area of about 120 to 130 m 2 . The network of capillaries is so dense that it may be thought of as a sheet of blood interrupted by small vertical supporting posts. The pulmonary veins that lie at the boundaries between lung units defined by bronchoarterial divisions return blood to the left atrium. By virtue of their larger numbers and thinner walls, the pulmonary veins provide a large reservoir for blood and help maintain a constant left ventricular output in the face of a variable pulmonary arterial flow.
The bronchial arteries (usually three originating directly or indirectly from the aorta, but variable in number) provide a source of well-oxygenated systemic blood to the lung’s tissues. This blood supply nourishes the walls of the bronchi and proximal bronchioles, larger blood vessels, and nerves in addition to perfusing the lymph nodes and most of the visceral pleura. There are numerous communications between the bronchial arterial system and the remainder of the pulmonary vascular bed: a portion of the blood returns to the right atrium via bronchial veins, and a portion drains into the left atrium via pulmonary veins. Although the bronchial arteries normally receive only 1% to 2% of the cardiac output, they hypertrophy in chronically infected lungs, and blood flow may easily increase by more than 10-fold. This is clinically important because virtually all hemoptysis originates from the bronchial vessels in disorders such as cystic fibrosis or other causes of suppurative bronchiectasis.
Histologically, the pulmonary arteries can be classified as elastic, muscular, partially muscular, or nonmuscular. The elastic pulmonary arteries are characterized by elastic fibers embedded in their muscular coat, whereas the smaller muscular arteries have a circular layer of smooth muscle cells bounded by internal and external elastic laminae. As arteries decrease further in size, only a spiral of smooth muscle remains (partially muscular arteries), which ultimately disappears so that vessels still larger than capillaries have no muscle in their walls (nonmuscular arteries). In the adult lung, elastic arteries are greater than 1000 µm in diameter, and muscular arteries are usually in the range of 250 µm. In the pediatric age group, histologic structure is not as easily determined from vessel size. During lung growth, a remodeling of the pulmonary vasculature occurs. Muscularization of the arteries lags behind multiplication of alveoli and appearance of new arteries. Therefore, the patient’s age must be considered before histologic structure can be assumed from vessel size within the pulmonary acinus ( Fig. 6.9 ). Notably, in the fetus and newborn, the amount of pulmonary arterial smooth muscle is increased. This is functionally important because high pulmonary arterial resistance is a feature of the fetal circulation in association with a large right to left shunt via the ductus arteriosus.

The endothelium of the pulmonary vascular system is continuous and nonfenestrated. It is an intensely active cell layer and is not just serving a passive barrier function. The endothelial cell produces a glycocalyx that interacts with blood-borne substances and blood cellular elements, thereby influencing such homeostatic functions as hemostasis. The endothelium produces von Willebrand factor, which is part of the factor VIII complex and is necessary for normal platelet function. Within endothelial cells, von Willebrand factor is stored in specific granules termed Weibel-Palade bodies. Similarly, there are enzymes located on the surface and within the endothelial cell itself that are capable of synthesizing, altering, or degrading blood-borne vasoactive products. The individual cells are separated by gaps of approximately 3.5 nm in radius, which allow the free movement of water and small ions but restrict the movement of proteins. The cells and the basement membrane on which they sit carry different net surface charges, which affect the movement of anionic macromolecules such as proteins and thus affect lung water and solute exchange (see Chapter 36 ). The capacity of the pulmonary endothelium and its basement membrane to restrict fluid and protein movement is impressive. It has been estimated that in the adult human, the amount of lung lymph flow is only 10 to 20 mL/h despite a total blood flow of 300,000 mL/h.
Lymphatic System
There is an extensive interconnecting network of lymphatic vessels throughout the lung. The major function of this network is to collect the protein and water that has moved out of the pulmonary vascular space and to return it to the circulation, thus maintaining the lung at an appropriate degree of hydration. The lymphatic vessels travel alongside the blood vessels in the loose connective tissue of the pleura and bronchovascular spaces. It is likely that there are no lymphatics within the alveolar wall itself and that juxta-alveolar lymphatics represent the beginning of the pulmonary lymphatic system. Histologically, the lymphatic capillaries consist of thin, irregular endothelial cells that lack a basement membrane. Occasionally, there are large gaps between endothelial cells that allow direct communication with the interstitial space. Larger lymphatic vessels contain smooth muscle in their walls that undergoes rhythmic contraction. This muscular contraction plus the presence of funnel-shaped, monocuspid valves ensures an efficient unidirectional flow of lymph. In addition to helping maintain lung water balance, the lymphatic system is one of the pulmonary defense mechanisms. It aids in removal of particulate matter from the lung, and aggregates of lymph tissue near major airways contribute to the host’s immune response.
Innervation of the Lung
The lung is innervated by both components of the autonomic nervous system. Parasympathetic nerves arise from the vagus nerve, and sympathetic nerves are derived from the upper thoracic and cervical ganglia of the sympathetic trunk. These branches congregate around the hila of the lung to form the pulmonary plexus. Myelinated and nonmyelinated fibers then enter the lung tissues and travel along with and innervate the airways and blood vessels. Although the anatomic location of pulmonary nerves has been elucidated, their physiologic role in health and disease is incompletely understood. In general, the airways constrict in response to vagal stimulation and dilate in response to adrenergic stimulation. The postnatal pulmonary vasculature appears to be maximally dilated under normal conditions, and it is difficult to demonstrate any significant physiologic effect of either parasympathetic or sympathetic stimulation. The vascular response, however, is influenced by age and initial vascular tone. For example, in fetal lungs where there is an abundance of pulmonary vascular smooth muscle, vagal stimulation results in significant vasodilation, and sympathetic stimulation results in marked vasoconstriction.
Sensory nerves from the lungs are vagal in origin and arise from slowly and rapidly adapting receptors and from C-fiber receptors. The slowly adapting (stretch) receptors, located in the smooth muscle of the airway, are stimulated by an increase in lung volume or transpulmonary pressure. They induce several physiologic responses including inhibition of inspiration (Hering-Breuer reflex), bronchodilation, increased heart rate, and decreased systemic vascular resistance. The rapidly adapting vagal (irritant) receptors are activated by a wide variety of noxious stimuli, ranging from mechanical stimulation of the airways to anaphylactic reactions within the lung parenchyma. The rapidly adapting receptors induce hyperpnea, cough, and constriction of the airways and larynx. C-fiber receptors are the terminus of nonmyelinated vagal afferents. They include the J receptors that are located near the pulmonary capillaries and are stimulated by pulmonary congestion and edema; they evoke a sensation of dyspnea and induce rapid, shallow breathing along with laryngeal constriction during expiration.
In addition to the sympathetic and parasympathetic nervous systems, humans and several other species have a third nervous system within their lungs. The noncommittal name nonadrenergic noncholinergic nervous system has been chosen because its function and properties are not understood. Purines, substance P, and vasoactive intestinal polypeptide have been suggested as possible neurotransmitters for this system.
Interstitium
The interstitium plays several roles in lung function in addition to providing a structural framework that consists of insoluble proteins. The ground substance influences cell growth and differentiation and lung water and solute movement. The cells contained within the interstitial region of the lung not only play individual roles that result from their contractile or synthetic properties, but they also interact with other cells (e.g., the endothelium and epithelium) to alter the basic structure and function of the lung.
A continuous fiber scaffold is present in the interstitium with an axial system (along the airways from the hilum to the alveolar ducts), a peripheral system (along the visceral pleura into interlobular septa), and a septal system (along the alveolar septa) ( Fig. 6.10 ). Most of the interstitial matrix of the lung is composed of type I collagen which, along with the less common collagen subtypes, forms a structural fibrous framework within the lung. Elastin provides elasticity and support to the structures. Both elastin and collagen turn over very slowly under normal conditions. However, rapid remodeling with changes in these proteins sometimes occurs. In diseases such as α 1 -antitrypsin deficiency (where neutrophil elastases degrade elastin) and pulmonary fibrosis (where there is increased amounts of collagen), there are marked qualitative and quantitative changes in these proteins. The remainder of the matrix is made up of proteoglycans and glycosaminoglycans. These carbohydrate-protein complexes can affect cell proliferation and differentiation in addition to their known effect on cell adhesion and attachment (e.g., laminin) and ability to diminish fluid movement (glycosaminoglycans).

Fibroblasts are capable of synthesizing components of the extracellular matrix (e.g., collagen, elastin, and glycosaminoglycans) and hence contribute significantly to the composition of the lung’s interstitial space. They can be found within all of the interstitial regions of the lung, although their apparent structure may change, emphasizing the heterogeneity of this cell population. For example, there are fibroblasts with contractile properties (myofibroblasts), some of which also contain lipid bodies in their cytoplasm (lipofibroblasts), although lipofibroblasts are not a common cell type in the human lung. Similarly, it is likely that morphologically similar fibroblasts are not similar in terms of proliferative capacity and ability to synthesize various types of collagen. Data suggest that fibrotic lung diseases are characterized by the loss of the normal heterogeneous fibroblast population and that there may be a selection for certain clones that promote inappropriate focal collagen deposition within the lung parenchyma.
Smooth muscle cells influence the bronchomotor and vasomotor tone within the conducting airways and blood vessels. They are also seen within the free edge of the alveolar septa together with elastic fibers where they form an alveolar entrance ring that is capable of constricting or dilating. The smooth muscle cells form bundles connected by nexus or gap junctions that enable electrical coupling and synchronous contraction. The pericyte is another contractile interstitial cell that is found embedded in the endothelial basement membrane. It is believed to be a precursor cell that can differentiate into other cell types such as mature vascular smooth muscle cells.
There are a variety of interstitial cells that are concerned with innate and adaptive defense of the lung. The interstitial macrophage and the alveolar macrophage are major effectors of the innate immune system, which they manage by ingesting particulate matter and removing it from the lung or by processing proteins or other antigens for presentation to adaptive immune cells. These macrophages are capable of secreting many compounds, including proteases and cytokines (substances capable of modulating the growth and function of other cells). B and T lymphocytes, including subsets such as T-regulatory cells (T-reg), are present in the lung and especially within the bronchus-associated lymphoid tissue (BALT), where they contribute to the humoral and cellular-mediated immune response. Further details on the innate and adaptive immune system are available in Chapter 8 .
Although not within the interstitium per se, there are large numbers of intravascular cells such as granulocytes that adhere to the pulmonary endothelium. Indeed, next to the bone marrow and spleen, there are more granulocytes within the lung than in any other organ. These granulocytes can be released into the systemic circulation during such stimuli as exercise or the infusion of adrenalin, and this demargination is responsible for the concomitant blood leukocytosis. These leukocytes are also in a prime location for movement into the lung should an infection or inflammatory stimulus occur. There is much evidence to suggest that the pulmonary granulocyte contributes to the pulmonary dysfunction seen in acute lung injury or acute RDS. Leukocytes also contain proteases that are thought to play a role in the development of emphysema and in the lung destruction that occurs in cystic fibrosis.
Growth and Development of the Lung
Prenatal Lung Growth
Lung development has been divided into various stages with names that reflect the respective histological appearance of the lung, the region of the lung that is most obviously developing, or both. The literature quotes different ranges for the different stages of human lung development. This concept is reinforced by the “overlap” between the different stages indicated in Fig. 6.11 . However, the available data contrasts with the mouse where at least the airway branching pattern is remarkably stereotypical in that all fetuses develop along the same timeline. It may be that there are inadequate data for the human fetal lung to detect this stereotypical development during the longer gestation or that, as with all developmental profiles, each individual human fetus develops along its own timeline. Prenatal lung growth and development is discussed in greater detail in the literature and in Chapter 2 .

The embryonic stage is the first stage of human fetal lung development and takes place from approximately 3 to 6 weeks’ gestational age (GA). The lung first appears as a ventral outpouching of the primitive gut. The primary bronchi elongate into the mesenchyme and divide into the two main bronchi. Another key event is that the main pulmonary artery arises from the sixth pharyngeal arch. Congenital abnormalities of the lung may occur during this stage (e.g., lung agenesis, tracheobronchial fistula).
The pseudoglandular stage occurs from approximately 6 to 16 weeks’ GA. During this period, airway branching continues and the mesenchyme differentiates into cartilage, smooth muscle, and connective tissue around the epithelial tubes. By the end of the pseudoglandular period, all major conducting airways, including the terminal bronchioles, have formed. Arteries are evident alongside the conducting airways, and by the end of the pseudoglandular period, all preacinar arterial branches have formed. Congenital abnormalities of the lung may occur during this stage (e.g., bronchopulmonary sequestration, cystic adenomatoid malformation, and congenital diaphragmatic hernia).
The canalicular stage occurs from approximately 16 to 26 weeks’ GA, and during that time, the respiratory bronchioles develop. By the end of this stage, each ends in a terminal sac (also termed a saccule ). The glandular appearance is lost as the interstitium has less connective tissue and the lung develops a rich vascular supply that is closely associated with the respiratory bronchioles.
The saccular stage occurs from approximately 26 to 36 weeks’ GA. During this period, significant capillary proliferation and thinning of the epithelium permits close contact between the airspace and the bloodstream, thus enabling gas exchange. Elastic fibers, which will be important in subsequent true alveolar development, begin to be laid down. At this time, cuboidal (type II) and thin (type I) epithelial cells begin to line the airspace.
The alveolar period commences at approximately 36 weeks’ GA. Secondary septa form on the walls of the saccules and grow into the lumen forming the walls of true alveoli.
Distention of the lungs’ airspaces by fetal lung liquid is essential for normal lung development. This fluid is neither a mere ultrafiltrate of plasma nor aspirated amniotic fluid. Rather it is generated by the epithelium’s active secretion of chloride into the developing lung’s lumen with sodium and water following passively ( Fig. 6.12A ). An inadequate amount of fetal lung liquid is associated with lung hypoplasia.

Congenital abnormalities of the lung may occur during the various stages. In addition, factors such as oligohydramnios or decreased fetal breathing may interfere with the development of the distal lung unit, including the development of alveoli. In contrast, if there is obstruction to outflow of tracheal fluid, as occurs in laryngeal atresia, there is pulmonary hyperplasia.
The Lung at Birth
Many dramatic changes must occur in the lungs during the transition from intrauterine to extrauterine life. The lung’s epithelium must change from fluid secretion to fluid absorption, the distal lung units must fill with and retain inhaled air, and blood flow must increase approximately 20-fold.
At the time of birth, the lungs contain approximately 30 mL/kg of fetal lung liquid. Approximately of this fluid is squeezed out during a vaginal delivery, but all of the fluid remains in the airspaces in an infant born by cesarean section. Thus, fetal lung liquid secretion must either greatly decrease or cease totally, and the fluid must be removed. Catecholamines released during labor can temporarily convert the fetal lung from a fluid-secreting organ to a fluid-absorbing organ by initiating the active transport of sodium by the distal lung epithelium (see Fig. 6.12B ). The clearance of fetal lung liquid from the newborn’s airspaces takes many hours, and the increase in oxygen tension at the time of birth is one key factor that permanently converts the lung epithelium into a sodium-absorbing mode.
In utero, little blood flows through the lung despite a relatively high perfusion pressure. This is because of the abundance of pulmonary vascular smooth muscle and the vasoconstrictor effect of the low fetal partial pressure of oxygen (P o 2 ) (<30 mm Hg). Although during the last trimester, concomitant with surfactant production, blood flow increases to 7% of the cardiac output, it is only at birth that marked increases in the capacity and distensibility of the pulmonary vasculature occur. Several mechanisms are responsible for the changes in circulation. Inflation of the lung with air results in mechanical distention of the vessels, and improvement in oxygenation removes hypoxic vasoconstriction. In addition, the increase in partial pressure of oxygen in arterial blood (Pa o 2 ) and changes in flow result in the release of mediators that contribute to the vasoconstriction of the ductus arteriosus and umbilical vessels and dilation of the pulmonary vascular bed. After birth the vessels dilate, which allows the necessary blood flow to the lungs, and the measured wall/lumen ratio decreases. After about 10 days of extrauterine life, the lumina are wider, regardless of the GA of the baby.
Postnatal Lung Growth
Postnatal lung growth continues throughout infancy and childhood and into the adolescent years. Throughout life, the average values of lung lobe weight expressed as a percentage of total lung weight are approximately: right upper lobe 20%, right middle lobe 8%, right lower lobe 25%, left upper lobe 22%, and left lower lobe 25%. Throughout the pediatric years, the tracheal diameter approximately triples, and the airways increase in their cross-sectional diameter ( Fig. 6.13 ). Excised human lung studies suggest that this growth occurs until around 5 years of age and is associated with marked changes in the relative conductance of central versus more peripheral airways ( Fig. 6.14 ).


Most postnatal lung growth involves the acinar area. New secondary septa continue to appear on the walls of the saccules and grow into the airspace, thus creating more true alveoli. Alveoli continue to increase in number through segmentation of these primitive alveoli and through transformation of terminal bronchioles into respiratory bronchioles, the latter being a process known as alveolarization. Alveolar dimensions and number both increase as the lungs and body grow with the internal surface area of the lung paralleling body mass (~1 to 1.5 m 2 /kg of body weight). It is agreed that alveoli appear prior to birth, and various studies have suggested that there are approximately 20 to 150 million alveoli at birth. A morphometric study of the lungs of 56 infants and children who died suddenly or after a brief illness indicates that there are approximately 200 to 300 million alveoli by the end of the second year of life, at which time alveolar multiplication slows markedly. After 2 years of age, boys have a larger number of alveoli and alveolar surface area than girls regardless of age and stature. Although recent observations suggest that alveolarization continues during adolescence, very few new alveoli develop after 8 years of age. Animal and human case studies suggest that the adult lung can, under certain circumstances, develop a small number of new alveoli. After alveolar multiplication stops, further growth of the airspace occurs through an increase in alveolar dimensions. In the mature adult lung, the final number of alveoli averages 480 million with individual subjects varying from 200 million to 800 million. The final number is related to total lung volume. An individual alveolus is 200 to 300 µm in diameter. As alveolar multiplication occurs, new blood vessels appear within the acinus. This explains the commensurate increase in the single breath diffusing capacity for carbon monoxide (CO) as the lungs grow.
Prenatal (saccular) walls, as well as secondary septa that form postnatally during the alveolar period, contain a double-capillary network. The adult lung, however, contains a single capillary layer interwoven with a sheet of septal connective tissue. The phase of remodeling the septal capillary bilayer into a single layer (microvascular maturation) occurs from early after birth up to the age of about 2 to 3 years.
Healthy children grow along their lung function growth curve similar to how children grow along their own height curve. For example, if a healthy child is born with lung volumes at the 10th percentile, he or she will usually maintain this status throughout childhood. It is known that the reference equations for lung function measurements must be adjusted for African-American children, since they have lower lung function for a given height compared to Caucasian children. Indeed, genetic analyses have shown that the forced expiratory flow in 1 second (FEV 1 ) and forced vital capacity (FVC) are related to the number of ancestral markers related to African ancestry.
When the structure and mechanical behavior of the young infant’s and child’s respiratory system are compared to those of the mature, but not the elderly, adult, important differences emerge that are likely to influence the pattern of disease. Some of these differences, such as reduced lung recoil, are shared by the infant and elderly and likely influence the pattern of respiratory disease in both populations. The young lung lacks elastic recoil, because elastin is still being created; as a result, airways are less well supported, and there is greater airway closure; this favors inhomogeneity of gas exchange and the development of patchy atelectasis. The elderly have low lung recoil, because they have lost elastin through the aging process, and there is greater loss of recoil when elastin degradation occurs by mechanisms promoting emphysema. The chest wall is relatively more compliant in the young child and stiffens with increasing age. As a result, the infant can develop paradoxical respiration. Respiratory muscle activation during inspiration can produce inward displacement of the rib cage, contributing to increased respiratory work for a given level of ventilation, particularly during rapid eye movement (REM) sleep. The deformability of the chest wall influences findings on physical examination. Chest wall–abdominal paradox may be normal in the premature infant during REM sleep but not in the older child or adult.
Postnatal lung growth can be impaired by restriction of the lung (e.g., in kyphoscoliosis) or augmented (e.g., in remaining lung post pneumonectomy). Lung capacities and airway flows continue to increase until late adolescence. Once adult life is achieved, then nonsmoking men and women have an annual decline in their FEV 1 of approximately 20 mL/year. The rate of decline during adult life is increased when individuals smoke or have a history of repeated childhood respiratory disorders.
Ventilation and Mechanics of Breathing
The principal function of the lung is to perform gas exchange, that is, to enrich the blood with oxygen and cleanse it of carbon dioxide. An essential feature of normal gas exchange is that the volume and distribution of ventilation are appropriate. Ventilation of the lung depends on the adequacy of the respiratory pump (muscles and chest wall) and the mechanical properties of the airways and gas-exchanging units.
It is traditional and useful to consider mechanical events as belonging to two main categories: the static-elastic properties of the lungs and chest wall and the flow-resistive or dynamic aspects of moving air. Changes in one category may be associated with compensatory changes in the other. Thus, many diseases affect both static and dynamic behavior of the lungs. Often, the principal derangement is in the elastic properties of the tissues or in the dimensions of the airways, and the treatment or alleviation of symptoms depends on distinguishing between them.
Before we discuss the mechanical aspects of lung function and gas exchange, it is important to review several basic physical laws concerning the behavior of gases and also the related abbreviations and symbols that will be used.
Definitions and Symbols
The principal variables for gases are as follows:
V = gas volume
V̇ = volume of gas per unit time
P = pressure
F = fractional concentration in dry gas
R = respiratory exchange ratio, carbon dioxide/oxygen
f = frequency
D l = diffusing capacity of lung
The designation of which volume or pressure is cited requires a small capital letter after the principal variable. Thus, V o 2 = volume of oxygen; P b = barometric pressure.
i = inspired gas
e = expired gas
a = alveolar gas
t = tidal gas
d = dead space gas
b = barometric pressure
When both location of the gas and its species are to be indicated, the order is V io 2 , which means the volume of inspired oxygen.
stpd = standard temperature, pressure, dry (0° C, 760 mm Hg)
btps = body temperature, pressure, saturated with water vapor
atps = ambient temperature, pressure, saturated with water vapor
The principal designations for blood are as follows:
S = percentage saturation of gas in blood
C = concentration of gas per 100 mL of blood
Q = volume of blood
Q̇ = blood flow per minute
a = arterial
= mixed venous
c = capillary
All sites of blood determinations are indicated by lowercase initials. Thus, Pa co 2 = partial pressure of carbon dioxide in arterial blood; = partial pressure of oxygen in mixed venous blood; and Pa o 2 = partial pressure of oxygen in a capillary.
The measurement of pressures can be confusing because pressure can be expressed using different units. For example, atmospheric pressure at sea level can be expressed by many seemingly different, but similar, values: it is important to remember the following:
1 atmosphere = 100 kilopascals ( kPa ) = 760 mm Hg = 760 Torr
The unit Torr is named after Evangelista Torricelli, who discovered the principle of the barometer and used the height of a column of mercury to measure pressures; for general use, one can use the following equation:
1 mm Hg = 1 Torr
Both pleural space and ventilator pressures are typically described as cm H 2 O, since a water-filled manometer was the initial tool used to measure these pressures; it was very hard to measure these low pressures by looking at only millimeter changes in the height of a column of mercury. Care must be taken when reviewing the literature on pulmonary vascular pressures as they can be expressed in either mm Hg or cm H 2 O. The conversion from cm H 2 O to mm Hg is as follows:
1 cm H 2 O = 0.736 mm Hg
Properties of Gases
Gases behave as an enormous number of tiny particles in constant motion. Their behavior is governed by the gas laws, which are essential to the understanding of pulmonary physiology.
Dalton’s law states that the total pressure exerted by a gas mixture is equal to the sum of the pressures of the individual gases. The pressure exerted by each component is independent of the other gases in the mixture. For instance, at sea level, air saturated with water vapor at a temperature of 37°C has a total pressure equal to the atmospheric or barometric pressure with the partial pressures of the components as follows:
PB = 760 mm Hg = PH 2 O ( 47 mm Hg ) + PO 2 ( 149.2 mm Hg ) + PN 2 ( 563.5 mm Hg ) + PCO 2 ( 0.3 mm Hg )
The gas in alveoli contains 5.6% carbon dioxide, BTPS. If P b = 760 mm Hg, then,
PACO 2 = 0.056 ( 760 − 47 ) = 40 mm Hg .
Boyle’s law states that at a constant temperature, the volume of any gas varies inversely as the pressure to which the gas is subjected since pressure times volumes is a constant. Because respiratory volume measurements may be made at different barometric pressures, it is important to know the barometric pressure and to convert to standard pressure, which is considered to be 760 mm Hg.
Charles’ law states that if the pressure is constant, the volume of a gas increases in direct proportion to the absolute temperature. At absolute zero (−273°C), molecular motion ceases. With increasing temperature, molecular collisions increase so that at constant pressure volume must increase.
In all respiratory calculations, water vapor pressure must be taken into account. The partial pressure of water vapor increases with temperature but is independent of atmospheric pressure. At body temperature (37°C), fully saturated gas has a P h 2 o of 47 mm Hg.
Gases may exist in physical solution in a liquid, escape from the liquid, or return to it. At equilibrium, the partial pressure of a gas in a liquid medium exposed to a gas phase is equal in the two phases. Note that in blood, the sum of the partial pressures of all the gases does not necessarily equal atmospheric pressure. For example, in venous blood, P o 2 has fallen from the 100 mm Hg of the arterial blood to 40 mm Hg, while partial pressure of carbon dioxide (P co 2 ) has changed from 40 to 46 mm Hg. Thus, the sum of the partial pressures of O 2 , CO 2 , and N 2 in venous blood equals 655 mm Hg. This provides the physiologic reason why patients who experience a pneumothorax can eventually reabsorb their pneumothorax and do so more rapidly if they inhale gases with an F I O 2 > 0.21.
Elastic Recoil of the Lung
The lung is an elastic structure that tends to decrease its size at all volumes. The elasticity of the lung depends on the structural components, the geometry of the terminal airspaces, and the presence of an air-liquid interface. When a lung is made airless and is then inflated with liquid, the elastic recoil pressure at large volumes is less than half that of a lung inflated to the same volume with air ( Fig. 6.15 ). Thus, the most significant determinant of the elastic properties of the lung is the presence of an air-liquid interface.

The increase of elastic recoil in the presence of an air-liquid interface results from the forces of surface tension. What is surface tension? When molecules are aligned at an air-liquid interface, they lack opposing molecules on one side. The intermolecular attractive forces are then unbalanced, and the resultant force tends to move molecules away from the interface. The effect is to reduce the area of the surface to a minimum. In the lungs, the forces at the air-liquid interface operate to reduce the internal surface area of the lung, and thus they augment elastic recoil. A remarkable property of the material at the alveolar interface, the alveolar lining layer containing pulmonary surfactant, is its ability to achieve a high surface tension at large lung volumes and a low surface tension at low volumes. Surfactant is a phospholipid-protein complex that when compressed forms insoluble, folded-surface films of low surface tension. The ability to achieve a low surface tension at low lung volumes tends to stabilize the airspaces and prevent their closure.
The exact method of lung stabilization and the concomitant role of surfactant in this stabilization can be debated. The classic interpretation is that without surfactant, the smaller alveoli would tend to empty into the larger alveoli in accordance with the Laplace relationship, which relates the pressure across a surface (P) to surface tension (T) and radius (r) of curvature. For a spherical surface, P = 2T/r. The smaller the radius, the greater is the tendency to collapse. The difficulty with this hypothesis is that the individual lung units are drawn as independent but communicating bubbles or spheres ( Fig. 6.16A ). This is not representative of structure of the lung because the alveolar walls are planar and not spherical. In addition, the inside wall of one alveolus is the outside wall of the adjacent alveolus. This last explanation has been utilized to develop the interdependence model of lung stability, which indicates that surface and tissue forces interact to maintain the lungs’ inherent structure with the fibrous components playing an important role (see Fig. 6.16B ).

The elastic recoil of the lung is responsible for the lung’s tendency to pull away from the chest wall with the resultant subatmospheric pressure in the pleural space. Lung recoil can therefore be derived from measurement of the pleural pressure when no air flow is occurring and alveolar pressure is zero. (The pressure measurement is taken with the patient holding his or her breath for a brief period with the glottis open.)
The pressure within the esophagus can be used as an index for mean pleural pressure. This is a reasonable assumption as long as there is no paradoxical rib cage movement. However, it is not a reasonable assumption for premature infants, term infants in REM sleep, and older infants with severe lung disease. For these infants, no average pleural pressure exists, and calculations of resistance and compliance will not be accurate using this method. When pleural pressure is estimated with an esophageal balloon, one must be careful to avoid artifacts resulting from the gravitational pressure of the mediastinum. For this reason, these measurements are best performed with the patient in the upright or lateral rather than the supine position. Once a series of pressure measurements has been made during brief breath-holds at different lung volumes, a pressure-volume curve of the lung can be constructed ( Fig. 6.17 ).

Compliance of the Lung
The pressure-volume curve of the lung describes two measurements of the elastic properties of the lung: elastic recoil pressure and lung compliance. Elastic recoil pressure is the pressure generated at a given lung volume, whereas compliance is the slope of the pressure-volume curve, or the volume change per unit of pressure:
Compliance = Δ volume Δ pressure = L cm H 2 O
Compliance depends on the initial lung volume from which the change in volume is measured and the ventilatory events immediately preceding the measurement as well as the properties of the lung itself. At large lung volumes, compliance is lower, because the lung is nearer its elastic limit. If the subject has breathed with a fixed tidal volume (V t ) for some minutes, portions of the lung are not participating in ventilation, and compliance may be reduced. A few deep breaths, with return to the initial volume, will increase compliance. Thus, a careful description of associated events is required for correct interpretation of the measurement.
Changes in total lung compliance occur with age for two reasons; the lung’s elastic recoil increases during childhood prior to declining during later adult life, and the smaller the subject, the smaller is the change in volume for the same change in pressure. For example, ΔV/ΔP is close to 6 mL/cm H 2 O in infants, and is 125 to 190 mL/cm H 2 O in adults. It is more relevant to a description of the elastic properties of the lung to express the compliance in relation to a unit of lung volume such as the functional residual capacity (FRC). When this is done, the compliance of the lung/FRC, or the specific compliance, changes much less. It is worth reemphasizing that total lung compliance is a function not only of the lung’s tissue and surface tension characteristics but also of its volume. This is especially important to remember when compliance has been measured in newborn infants with RDS. The total compliance is a composite of the lung’s elastic properties and the number of open lung units. In RDS, sudden changes in total measured compliance (if uncorrected for simultaneously measured lung gas volume) will predominantly, if not exclusively, reflect the opening and closing of individual lung units.
Lung compliance may also be measured during quiet breathing with pressure and volume being recorded at end-inspiration and end-expiration. The resultant value is the dynamic lung compliance. Although dynamic lung compliance does reflect the elastic properties of the normal lung, it is also influenced by the pressure required to move air within the airways. Therefore, dynamic lung compliance increases with increased respiratory rate and with increased airway resistance. Air flow is still occurring within the lung after it has ceased at the mouth, and pleural pressure reflects both the elastic recoil of the lung and the pressure required to overcome the increased airway resistance. Indeed, dynamic lung compliance can be used as a sensitive test of obstructive airway disease.
Elastic Properties of the Chest Wall
The chest wall is also an elastic structure, but in contrast to the lung, it tends to push outward at low volumes and inward at high volumes. For example, when air is introduced into the pleural space: the lung collapses and the chest wall springs outward.
Compliance of the chest wall can be measured by considering the pressure difference between the pleural space or esophagus and the atmosphere, per change in volume. Significant changes in thoracic compliance occur with age ( Fig. 6.18 ). In the range of normal breathing, the thorax of the infant is nearly infinitely compliant. The pressures measured at different lung volumes are about the same across the lung as those measured across lung and thorax together. The functional significance of the high compliance of the neonatal thorax is observed when there is lung disease. The necessarily greater inspiratory effort and more negative pleural pressure can “suck” in the chest wall, resulting in less effective gas exchange and a higher work of breathing.

With advancing age, the thorax becomes relatively stiffer. Changes in volume-pressure relations are profitably considered only if referred to a reliable unit, such as a unit of lung volume or a percentage of total lung capacity (TLC). Considered on a percentage basis, compliance of the thorax decreases with age. It remains unclear how much of this change is contributed by changes in tissue properties (e.g., increasing calcification of ribs and connective tissue changes) and how much is a disproportionate growth of the chest wall relative to the lung.
Lung Volumes
Definition
The partition of commonly used lung volumes can be understood by studying Fig. 6.19 . The spirogram on the left represents the volume of air breathed in and out by a normal subject. The first portion of the tracing illustrates normal breathing and is called the tidal volume (V t ). The subject then makes a maximal inspiration followed by a maximum expiration: the volume of expired air is the vital capacity (VC). The volume of air that still remains in the lung after a maximal expiration is the residual volume (RV), whereas the volume of air remaining in the lung after a normal passive expiration with relaxed respiratory muscles is the FRC. The maximum amount of air that a subject can have in the lungs is called the TLC. In healthy young subjects, TLC correlates best with the subject’s sitting height.

The volumes and capacities of the lungs are determined by many factors, including muscle strength, static-elastic characteristics of the chest wall and lungs, airway status, and patient age and cooperation. TLC is reached when the force generated by maximal voluntary contraction of the inspiratory muscles equals the inward recoil of the lung and chest wall. FRC occurs when the respiratory muscles are relaxed and no external forces are applied; it is therefore the volume at which the inward recoil of the lung is exactly balanced by the outward recoil of the chest wall (see Fig. 6.18 ).
Closing volume, the volume at which airways close in dependent regions of the lung, is not routinely measured but is important for our understanding of normal lung function (see: Distribution of Ventilation). Closing volume is graphically illustrated by the atelectasis observed in dependent regions of the infant lung in computed tomography scans of the chest.
In healthy children and young adults, end-expiratory lung volume during tidal volume breathing is equivalent to FRC. This is not the case in infants, who breathe at a lung volume higher than FRC. This higher volume seems to be a sensible solution to the infants’ problem of having an airway closing volume that exceeds FRC. An infant maintains the expiratory lung volume higher than FRC by a combination of postinspiratory diaphragmatic activity, laryngeal adduction, and rapid respiratory rate, which minimizes the time for expiration.
The factors determining RV vary with age. In adolescents and young adults, RV occurs when the expiratory muscles cannot compress the chest wall further. In young children and older adults, RV is a function of the patency of small airways and the duration of expiratory effort.
Measurement
Tidal volume and VC can be determined by measuring the expired volume. The measurement of FRC and RV requires another approach. Because both volumes include the air in the lungs that the patient does not normally exhale, they must be measured indirectly. One method uses the principle of dilution of the unknown volume with a known concentration of a gas that is foreign to the lung and only sparingly absorbed, such as helium. The patient breathes from a container with a known volume and concentration of helium in oxygen-enriched air. After sufficient time has elapsed for the gas in the lung to mix and equilibrate with the gas in the container, the concentration of helium in the container is remeasured. Because initial volume × initial concentration of helium = final volume × final concentration of helium, the final volume, which includes gas in the lungs, can be calculated.
The multiple breath inert gas washout method also can be used to calculate lung volumes such as FRC. In addition, it provides information regarding the lung clearance index (LCI) which is now recognized to be a sensitive indicator of peripheral airway dysfunction. It also has the advantage that it can be performed in uncooperative subjects such as infants and very young children (see Chapters 11 and 12 ).
Neither the helium dilution nor multiple breath inert gas washout methods can measure gas behind closed airways (“trapped gas”) or in regions of the lung that are poorly ventilated. There is, however, a method of measuring total gas volume within the thorax that depends on the change in volume that occurs with compression of the gas when breathing against an obstruction. Practically, this measurement requires the patient to be in a body plethysmograph and to pant against a closed shutter. The change in pressure can be measured in the mouthpiece; the change in volume can be recorded with a spirometer attached to the body plethysmograph:
V = P Δ V / Δ P
This method has the advantage of being able to be repeated several times per minute. It has the disadvantage of including some abdominal gas in the measurement.
There have also been concerns about the validity of the plethysmographic technique in patients with severe obstructive lung disease. This issue has not yet been resolved because the technique has been reported to overestimate the lung volume in adults but underestimate the lung volume in infants with obstructive lung disease.
Interpretation
Similar to body growth percentiles, there is a wide range of normal values for lung volumes. For example, the mean TLC for a child 140 cm tall is 3.2 L; however, the statistical range of normal (mean ± 2 SD) is 1.9 to 4.3 L. This range of normal values, when expressed as percentage predicted, is even greater for younger children or smaller lung volumes (such as RV). Owing to this wide range of normality, care must be exercised in the interpretation of lung volumes. Measurement of lung volumes is of greatest benefit when repeated over several months to assess the progress of a chronic respiratory illness and the efficacy of treatment. Healthy children grow along their lung function growth curve, much like they grow along their growth percentiles.
The VC is one of the most valuable measurements that can be made in a functional assessment, because it is highly reproducible and has a relatively narrow range of normal values. It can be decreased by a wide variety of disease processes, including muscle weakness, loss of lung tissue, obstruction of the airway, and decreased compliance of the chest wall. VC is therefore not a useful tool to discriminate between types of lesions. Its chief role is to assign a value to the degree of impairment and to document changes that occur with therapy or time. To decide whether obstructive or restrictive lung disease is present, it is useful to measure expiratory flow rates (see Chapters 11 and 12 ) and to observe the pattern of abnormalities in the other lung volumes. In obstructive lung disease (e.g., asthma), the smallest lung volumes are affected first; RV increases owing to abnormally high airway resistance at low lung volumes (air trapping), and as the disease progresses, the FRC increases. Although the increase in FRC (hyperinflation) may rarely be due to loss of lung recoil, the overdistention is usually compensating for partial lower airway obstruction. When the lung volume is increased, intrathoracic airways enlarge, and widespread partial obstruction may be partially relieved by the assumption of a larger resting lung volume. Whereas the TLC is only rarely affected in obstructive disease (e.g., asthma) in children, TLC and VC are the first lung volumes to be affected in restrictive diseases of the chest wall (e.g., kyphoscoliosis) or lung (e.g., pulmonary fibrosis).
Regional Lung Volumes
During normal breathing, different areas of the lung have different regional lung volumes; the upper airspaces are inflated more than the lower airspaces. Because static-elastic properties are fairly constant throughout the lung, these different regional lung volumes result from the gradient of pleural pressure that exists from the top to the bottom of the lung. Although gravitational forces are thought to be largely responsible, the mechanisms responsible for this pleural pressure gradient are incompletely understood. In the erect young adult lung, the pleural pressure is −8 cm H 2 O at the apex and only −2 cm H 2 O at the base. The significance of this phenomenon is that when a subject breathes in, the lowermost lung units will receive the majority of the inspired air ( Fig. 6.20 ). This is advantageous because the majority of pulmonary blood flow also goes to the base of the lung, and thus, blood flow and ventilation patterns are more closely matched.

Dynamic (Flow-Resistive) Properties of the Lung
Gas Flow Within Airways
The respiratory system must perform work to move gas into and out of the lungs. Because air moves into the lungs during inspiration and out of the lungs during expiration, and because the velocity of air flow increases from small airways to large airways, energy must be expended to accelerate the gas molecules. The respiratory system’s resistance to acceleration (inertance) is minimal during quiet breathing and will not be considered further. However, inertance becomes quite significant during very high breathing rates, as occurs in high-frequency ventilation. During quiet breathing, frictional resistance to air flow accounts for one-third of the work performed during quiet breathing. The magnitude of pressure loss due to friction is determined by the pattern of flow. Flow may be laminar (streamlined) or turbulent, and which pattern exists depends on the properties of the gas (viscosity, density), the velocity of air flow, and the radius of the airway. In general, there is laminar flow in the small peripheral airways and turbulent flow in the large central airways.
The laws governing the frictional resistance to flow of gases in tubes apply to pulmonary resistance. The equation for calculating the pressure gradient required to maintain a laminar flow of air through a tube is given by Poiseuille’s law:
P = V ˙ ( 8 l η π r 4 )
where P = pressure, V̇ = flow, l = length, r = radius of the tube, and n = the viscosity of the gas. The viscosity of air is 0.000181 poise at 20°C, or only 1% that of water. Because resistance = pressure/flow, it is clear that the most important determinant of resistance in small airways will be the radius of the tube, which is raised to the fourth power in the denominator of the equation.
The pressure required to maintain turbulent flow is influenced by airway diameter and gas density and is proportional to the square of the gas velocity. The effect of gas density on turbulent flow has both therapeutic implications. Children with viral laryngotracheobronchitis have marked narrowing of the subglottic area, which greatly increases the resistance to air flow. The pressure required to overcome this increased resistance in the large airways, and hence the work of breathing can be decreased by administering a low-density gas mixture (70% helium, 30% oxygen).
Measurement of Resistance
Resistance (R) is calculated from the equation:
R = driving pressure a i r flow
The pressure is measured at the two ends of the system, in the case of the lung, at the mouth and at the alveoli, and the corresponding flow is recorded. Measurement of alveolar pressure presents the greatest problem. Several methods have been used to measure alveolar pressure. The most common method employs a body plethysmograph. The subject sits in an airtight box and breathes through a tube connected to a pneumotachometer, an apparatus that measures air flow. When a shutter occludes the tube and air flow ceases, the mouth pressure is assumed to be equal to the alveolar pressure. Airway resistance can then be calculated because air flow, alveolar pressure, and ambient pressure are known.
Total pulmonary resistance can be measured in infants and children by the forced oscillation technique. This measurement includes airway resistance plus the tissue viscous resistance of the lung and chest wall. Nasal resistance is also included in the measurement if the infant is breathing through the nose. Although there are theoretical objections to this technique, it has several advantages. It does not require a body plethysmograph, estimates of pleural pressure, or patient cooperation, and it can be done quickly enough to be used on ill patients. A sinusoidal pressure applied at the upper airway changes the air flow, and the ratio of pressure change to flow change is used to calculate resistance. When the forced oscillations are applied at the so-called resonant frequency of the lung (believed to be 3 to 5 Hz), it is assumed that the force required to overcome elastic resistance of the lung and the force required to overcome inertance are equal and opposite so that all of the force is dissipated in overcoming flow resistance. This technique has demonstrated that infants with bronchiolitis have about a twofold increase in inspiratory pulmonary resistance and a threefold increase in expiratory resistance.
Several new techniques have been developed that are capable of measuring lung function in infants and young children. Each has its advantages, underlying assumptions, and limitations, and these techniques are discussed in detail in Chapter 11 .
Sites of Airway Resistance
The contribution of the upper airway to total airway resistance is substantial. The average nasal resistance of infants by indirect measurements is nearly half of the total respiratory resistance, as is the case in adults. It is hardly surprising that any compromise of the dimensions of the nasal airways in an infant who is a preferential nose breather will result in retractions and labored breathing. Likewise, even mild edema of the trachea or larynx will impose a significant increase in airway resistance.
In the adult lung, about 80% of the resistance to air flow resides in airways greater than 2 mm in diameter. The vast number of small peripheral airways provides a large cross-sectional area for flow and therefore contributes less than 20% to the airway resistance. Thus, these airways may be the sites of disease that may severely impair ventilation of distal airspaces without appreciably altering the total airway resistance. In the infant lung, however, small peripheral airways may contribute as much as 50% of the total airway resistance, and this proportion does not decrease until about 5 years of age. Thus, the infant and young child are particularly severely affected by diseases that affect the small airways (e.g., bronchiolitis).
Factors That Affect Airway Resistance
Airway resistance is determined by the diameter of the airways, the velocity of air flow, and the physical properties of the gas breathed. The diameter is determined by the balance between the forces tending to narrow the airways and the forces tending to widen them. One of the forces tending to narrow the airways is exerted by the contraction of bronchial smooth muscle. The neural regulation of bronchial smooth muscle tone is mediated by efferent impulses through autonomic nerves. Sympathetic impulses relax the airways, and the parasympathetic impulses constrict them. Bronchi constrict reflexly from irritating inhalants (e.g., sulfur dioxide and some dusts); by arterial hypoxemia and hypercapnia; by embolization of the vessels; by cold; and by some vasoactive mediators (e.g., acetylcholine, histamine, bradykinin, and leukotrienes). They dilate in response to an increase in systemic blood pressure through baroreceptors in the carotid sinus and to beta sympathomimetic agents such as salbutamol (selective beta 2 agonist), isoproterenol (beta 1 and beta 2 agonist), and epinephrine (nonselective alpha and beta agonist). The large airways are probably in tonic contraction in health, because in unanesthetized adults, atropine or isoproterenol will decrease airway resistance.
Airway resistance changes with lung volume but not in a linear manner. Increasing the lung volume to above FRC only minimally decreases airway resistance. In contrast, as lung volume decreases from FRC, resistance rises dramatically and approaches infinity at RV. Although alterations in bronchomotor tone play a role, it is the decrease in lung elastic recoil as lung volume declines that is the predominant mechanism for the change in airway resistance. The recoil of the lung provides a tethering or “guy wire” effect on the airways that tends to increase their diameter. Children of different ages will have different airway resistances owing to the different sizes of their lungs. Therefore, the measurement of airway resistance or its reciprocal (airway conductance) is usually corrected by dividing the airway conductance by the simultaneously measured lung volume. The resultant specific airway conductance is remarkably constant regardless of the subject’s age or height.
Dynamic Airway Compression
During a forced expiration, both the pleural and the peribronchial pressures become positive and tend to narrow the airways; forces tending to keep airways open are the intraluminal pressure and the tethering action of the surrounding lung. During active expiration, however, the intraluminal pressure must decrease along the pathway of air flow from the alveoli to the mouth, where it becomes equal to atmospheric pressure. Therefore, at some point in the airway, intraluminal pressure must equal pleural pressure, the equal pressure point (EPP). Downstream from the EPP, pleural pressure exceeds intraluminal pressure and thus is a force that tends to narrow the airways. Indeed, during periods of maximum expiratory flow, pleural pressure exceeds the critical closing pressure of the airways, which become narrowed to slits. Despite the cartilaginous support of the larger airways, the membranous portion of the wall of the trachea and large bronchi invaginates under pressure to occlude the airways. Maximum flow under this circumstance is therefore determined by the resistance of the airways located upstream from the EPP, and the driving pressure is the difference between the alveolar pressure and the pressure at the EPP. In disease states in which there is increased airway resistance, the EPP moves toward the alveoli because of the greater intraluminal pressure drop. Thus, small airways are compressed during forced expiration with severe flow limitation. With the measurement of pressure-flow and flow-volume curves during forced expiration, it is possible to calculate resistance upstream and downstream from the point of critical closure, or EPP. Increasing the lung volume increases the tethering action of the surrounding lung on the airways, and therefore close attention must be paid to the lung volume at which resistance measurements are made during these studies.
Work of Breathing
Work is defined as the force over distance or three-dimensionally as pressure during changes in volume. Thus, the work performed by the respiratory pump is defined by the volume changes of the lungs when the respiratory muscles generate a given pressure. The volume-pressure relationships of the respiratory system depend on properties of the lung and chest wall tissues or the ease with which the airways allow the passage of air. A substantial portion of the pressure generated by the respiratory muscles is applied to produce reversible rearrangements of the structure of the alveolar gas-liquid interface and the fibrous network of the lungs. Another large portion of the effort of the respiratory muscles is directed at producing rearrangements or interactions that are not reversible. The energy spent in such an effort is directly transformed into heat, which is then dissipated into the atmosphere or carried away by the circulating blood. The magnitudes of the work and the pressures derived from these processes generally bear a relationship to the rate of gas flow in and out of the lungs. In this regard, the respiratory system exhibits a resistive behavior for which the driving pressure determines the flow of air. Both the elastic and the resistive components of the work of breathing are usually increased in children with respiratory disease.
Respiratory work normally accounts for about 3% of an individual’s total oxygen consumption. This work is increased in various diseases, and establishing a diagnosis and formulating a therapy in these patients is almost always simplified when the clinician distinguishes between conditions that affect primarily the elastic (restrictive respiratory disease) and resistive (obstructive respiratory disease) behaviors of the respiratory system.
Distribution of Ventilation
The distribution of ventilation is influenced by several factors in the normal lung. The pleural pressure gradient results in a greater amount of the tidal volume going to the dependent areas of the lung (see Fig. 6.20 ). In addition, the rate at which an area of the lung fills and empties is related to both regional airway resistance and compliance. A decrease in an airway’s lumen increases the time required for air to reach the alveoli; a region of low compliance receives less ventilation per unit of time than an area with high compliance. The product of resistance and compliance (the “time constant”) is approximately the same in health for all ventilatory pathways. The unit of this product is time. Note the following:
Resistance = pressure flow = c m H 2 O L / s e c
and
Compliance = Δvolume ( L ) Δpressure ( c m H 2 O )
The product, then, is a unit of time, analogous to the time constant in an electrical system, which represents the time taken to accomplish 63% of the volume change.
As mentioned earlier in the chapter, peripheral airways contribute little to overall airway resistance after 5 years of age. However, in the presence of small airway disease, some areas of the lung have long-time constants, but those of others are normal. This is particularly evident as the frequency of respiration increases. With increasing frequency, air goes to those areas of the lung with short time constants. These areas then become relatively overdistended, and a greater transpulmonary pressure is required to inspire the same volume of air, because alveoli in these relatively normal areas are reaching their elastic limit. Thus, a decreased dynamic compliance with increasing frequency of respiration has been used as a test of small airway disease and indeed may be the only mechanical abnormality detectable in the early stages of diseases such as emphysema and cystic fibrosis.
Airway closure occurs in dependent areas of the lung at low lung volumes. The lung volume above RV at which closure occurs is called the closing volume. In infants, very young children, and older adults, airway closure occurs at FRC and therefore is present during normal tidal breathing. This results in intermittent inadequate ventilation of the respective terminal lung units and leads to abnormal gas exchange, notably to a lower Pa o 2 seen in these age groups.
Pulmonary Circulation
Physiologic Classification of Pulmonary Vessels
The pulmonary circulation is the only vascular bed to receive the entire cardiac output. This unique characteristic enables the pulmonary vascular bed to perform a wide variety of homeostatic physiologic functions. It provides an enormously large (~120 to 130 m 2 ) yet extremely thin surface for gas exchange, filters the circulating blood, controls the circulating concentrations of many vasoactive substances, and provides a large surface area for the absorption of lung liquid at birth. The nomenclature of the pulmonary vessels is at times confusing because the anatomic classification of the vessels often does not correspond to their physiologic role.
The anatomic and histologic characteristics of the pulmonary vasculature are described earlier in the chapter. It is important to understand that pulmonary vessels have been classified physiologically as extra-alveolar and alveolar vessels, fluid-exchanging vessels, and gas-exchanging vessels. When the outside of a vessel is exposed to alveolar pressure, it is classified as an alveolar vessel (capillaries within the middle of the alveolar septum), whereas extra-alveolar vessels (arteries, veins, and capillaries at the corner of alveolar septa) are intrapulmonary vessels that are subjected to a more negative pressure resulting from and approximating pleural pressure. The diameter of the extra-alveolar vessels is therefore greatly affected by lung volume, expanding as inspiration occurs. Although extra-alveolar vessels and alveolar vessels are subjected to different mechanical pressures, they are both classified as fluid-exchanging vessels because both leak water and protein and can contribute to the production of pulmonary edema. The anatomic location of gas-exchanging vessels is unclear but is likely limited to the capillaries and smallest arterioles and venules.
Pulmonary Vascular Pressures
The pressure within the pulmonary circulation is remarkably low considering that it receives the entire cardiac output (5 L/min in the adult human). Beginning a few months after birth, pulmonary arterial pressures are constant throughout life, with the average mean pulmonary arterial pressure being 15 mm Hg and the systolic and diastolic pressures being 22 and 8 mm Hg, respectively. The pulmonary venous pressure is minimally higher than the left atrial pressure, which averages 5 mm Hg. The pressure within human lung capillaries is unknown, but work in isolated dog lungs suggests it is 8 to 10 mm Hg, approximately halfway between the mean arterial and venous pressures. These values refer to pressures at the level of the heart in the supine position; because of gravity, pulmonary arterial pressures are near zero at the apex of the upright adult lung and close to 25 mm Hg at the base. Depending on their location, vessels have different pressures on their outside walls. As defined previously, the alveolar vessels are exposed to alveolar pressure, which fluctuates during the respiratory cycle, but will average out close to zero. In contrast, the extra-alveolar vessels are exposed to a negative fluid pressure on their outer walls, estimated to be between −6 and −9 cm H 2 O. The pressure on the outside of the pulmonary vessel is not a trivial matter, because the transmural pressure (inside pressure–outside pressure), rather than the intravascular pressure, is the pertinent hydrostatic pressure influencing vascular distention and the transvascular movement of water and protein (see Chapter 36 ).
Pulmonary Vascular Resistance
The resistance to blood flow through the lungs can be calculated by dividing the pressure across the lungs by the pulmonary blood flow.
R = mean P A pressure − mean L A pressure pulmonary blood flow
A decrease in resistance to blood flow can occur only through (1) an increase in the blood vessels’ lumenal diameters or (2) an increase in the number of perfused vessels. Each of these will contribute to an increase in the cross-sectional diameter of the pulmonary vascular bed. The diameter of an already open pulmonary vessel can be increased by decreasing the muscular tone of the vessel wall (e.g., with a vasodilating agent) or by increasing the transmural pressure (e.g., through increased pulmonary arterial or left atrial pressure). Previously unperfused pulmonary vessels may be opened up (“recruited”) when their transmural pressure exceeds their critical opening pressure. This occurs when intravascular pressures are raised or when a vasodilator has decreased the vessels’ critical opening pressure. An increase in cardiac output decreases the calculated pulmonary vascular resistance (PVR). This is important to remember when assessing vasodilating drugs; studies have been performed in which drugs were found to increase cardiac output substantially so that the calculated PVR falls. This decrease in resistance does not ensure that a particular drug has any direct vasodilating action at all, because the entire decrease in PVR may have resulted from its cardiac effects.
The interrelationship between lung volume and PVR is complex and is influenced by pulmonary blood volume, cardiac output, and initial lung volume. The principal reason for this complex relationship is that a change in lung volume has opposite effects on the resistances of the extra-alveolar and alveolar vessels. As the lung is inflated, the radial traction on the extra-alveolar vessels increases their diameter, whereas the same increase in lung volume increases the resistance to flow through the alveolar vessels (which constitutes 35% to 50% of the total PVR). It is reasonable to say, however, that PVR is at its minimum at FRC, and any change in lung volume (increase or decrease) will increase the PVR ( Fig. 6.21 ).

Active changes in PVR can be mediated by neurogenic stimuli, vasoactive compounds, or chemical mediators. The normal adult pulmonary circulation appears to be maximally dilated, since no stimulus has been found that can further dilate the pulmonary vessels. In contrast, the neonatal lung or the vasoconstricted adult lung vasodilates in response to a variety of agents, including nitric oxide, acetylcholine, β-agonist drugs, bradykinin, prostaglandin E, and prostacyclin.
The pulmonary circulation can undergo significant vasoconstriction, which is surprising in view of the paucity of muscle in postnatal lung vessels. Hypoxia is the most common potent pulmonary vasoconstricting agent. Hypoxic vasoconstriction, which occurs when the alveolar P o 2 falls below 50 to 60 mm Hg, is a local response independent of neurohumoral stimuli. Although many suggestions have been made, the exact mechanism of hypoxia-induced vasoconstriction is unknown. Acidosis acts synergistically with hypoxia to constrict the pulmonary vessels; however, it is unlikely that CO 2 alone has any direct effect on the pulmonary circulation in humans. Stimulation of the pulmonary sympathetic nerves results in a weak vasoconstrictive response in the dog lung but little or no response in the normal human adult pulmonary circulation. Vasoactive substances (e.g., histamine, fibrinopeptides, prostaglandins of the F series, and leukotrienes) are capable of constricting the pulmonary vascular bed. It had been believed that vasoconstriction in the pulmonary circulation took place predominantly, if not exclusively, within the arterial section of the vascular bed. However, it has been demonstrated that other regions of the bed may narrow in response to stimuli. For example, hypoxia can constrict the pulmonary venules of newborn animals and might increase resistance within the capillary bed by inducing constriction of myofibroblasts that are located within the interstitium of the alveolar-capillary membrane. The fetal and neonatal pulmonary circulation contains a large amount of smooth muscle, which enhances the response to vasoconstrictive stimuli.
Distribution of Blood Flow
Blood flow is uneven within the normal lung and is influenced by the vascular branching pattern and gravity that when standing results in more blood flow being directed to the dorsal caudal regions and less to the cephalad regions. Gravitational forces are largely responsible for the increasing flow from apex to base because the intravascular pressure of a given blood vessel is determined by the pulmonary arterial pressure immediately above the pulmonary valve and the blood vessel’s vertical distance from the pulmonary valve. Thus, with increasing height above the heart, the pulmonary arterial pressure decreases and less perfusion occurs. The opposite occurs for vessels located in the lung bases, and together these gravitational effects are responsible for a pressure difference of approximately 23 mm Hg between apical and basal pulmonary arteries.
These regional differences in lung perfusion are best understood in terms of West’s zones of perfusion ( Fig. 6.22 ). West’s zone I occurs when mean pulmonary arterial pressure is less than or equal to alveolar pressure, and as a result, no blood flow occurs (except perhaps during systole). Zone I conditions are present in the apices of some upright adults and result in unperfused yet ventilated lung units (alveolar dead space). Moving down from the lung apices, pulmonary arterial pressure becomes greater than alveolar pressure, with the latter being greater than venous pressure. These are zone II conditions, and blood flow is determined by the difference between arterial and alveolar pressures and is not influenced by venous pressure; an appropriate analogy would be that of a vascular “waterfall,” in which the flow rate is independent of the height of the falls. In zone III, left atrial pressure exceeds alveolar pressure, and flow is determined in the usual manner (i.e., by the arterial-venous pressure gradient).

Methods of Evaluating the Pulmonary Circulation
The chest radiograph is the most basic noninvasive and nonquantitative tool for determining the possible presence of pulmonary vascular disease. Prominence of the pulmonary outflow tract occurs when the elevated pressure distends the elastic main pulmonary arteries. Vascular markings may be increased or decreased; the former occurs when there is elevated pulmonary blood flow or volume without vascular remodeling, and the latter occurs when there is distal “pruning” of the vessel’s image when vascular remodeling has occurred. Computerized axial tomography and magnetic resonance imaging provide greater detail of the right ventricle and pulmonary vessels.
Echocardiography is able not only to assess the structure and function of the right ventricle but also to provide a reasonable estimate of right ventricular pressure by assessing the velocity of the small retrograde flow through the tricuspid valve that frequently occurs in significant pulmonary hypertension. Quantitative assessment of regional pulmonary blood flow can be made with intravenous injections of macroaggregates of albumin labeled with technetium 99m . The macroaggregates occlude a very small portion of the pulmonary vascular bed. The amount of regional blood flow can be determined by imaging the lungs with a large field-of-view gamma camera and determining the count rate with a computer. The perfusion lung scintigram can be combined with a ventilation scintigram performed with either a radioactive gas (e.g., xenon 133 or krypton 81m ) or a radiolabeled ( 99m Tc–diethylenetriamine-pentacetic acid) aerosol that is distributed like a gas.
Regional pulmonary angiography further delineates localized disturbance in blood flow, although the procedure requires cardiac catheterization. Direct measurements of pulmonary artery and pulmonary artery occlusion (“wedge”) pressures add further information. Occasionally, drugs can be infused into the pulmonary artery to evaluate the potential reversibility of pulmonary hypertension.
Further details regarding the pathobiology and treatment of pulmonary hypertension are available elsewhere and in Chapter 35 .
Muscles of Respiration
The importance of the muscles of respiration derives from the fact that these muscles, similar to the myocardium, can fail under abnormal circumstances and can induce or contribute to an impending or existing ventilatory failure.
The principal muscle of respiration is the diaphragm, a thin musculotendinous sheet that separates the thoracic from the abdominal cavity. In adults, its contraction causes descent of its dome and aids in elevation of the lower ribs, the latter referred to as the “bucket handle effect.” Some work indicates that the diaphragm has two separate but related functions. The costal part of the diaphragm (largely innervated by C5) acts to stabilize and elevate the lower rib cage during contraction. The vertebral (crural) portion (largely innervated by C3), a much thicker muscle, descends with contraction and is largely responsible for the volume change that occurs.
Three anatomic characteristics of the infant’s chest wall and diaphragm lead to decreased diaphragmatic efficiency and a lower fatigue threshold. First, the infant’s chest wall is highly compliant so that when there is respiratory disease there is increased work. Second, the diaphragm is less effective as a result of its higher position within the chest and less apposition to the rib cage. Third, the infant’s ribs are more horizontal, which lessens the bucket handle effect.
Other skeletal muscles located in the chest or abdominal wall (e.g., the intercostals, scalenes, and abdominal muscles) can play an important role in ventilation. During normal breathing, most of the accessory muscles are silent. However, during abnormal conditions or disease states, these muscles are recruited to stabilize the chest or abdominal wall so that the diaphragm may be more efficient. In addition, it has been demonstrated that the external intercostal muscles contract in acute asthmatic attacks not only during inspiration but also during expiration; this contraction maintains a higher lung volume and hence increases airway diameter. When airways are occluded during inspiration, abdominal muscles contract powerfully during expiration, pushing the abdominal contents and diaphragm toward the thoracic cavity. This action lengthens diaphragmatic fibers and enhances the capability of the diaphragm to generate force during the subsequent inspiration (length-tension curve).
The upper airways must be kept patent during inspiration, and therefore, the pharyngeal wall muscles, genioglossus, and arytenoid muscles are properly considered muscles of respiration. There is an increase in neural output to these muscles immediately before diaphragmatic contraction during inspiration. The newborn also contracts these muscles during expiration to provide an expiratory outflow resistance and thus keeps end-expiratory volume greater than the FRC.
Respiratory muscles, whether the diaphragm, upper airway, intercostal, or abdominal muscles, are not homogeneous muscles in terms of their cellular structure, blood supply, metabolism, and recruitment patterns. Adult mature skeletal muscles have a mixture of fibers, and respiratory muscles are no different. The adult diaphragm, for instance, is made of fast- and slow-twitch fibers. Slow-twitch fibers are oxidative, and fast-twitch fibers are either glycolytic or moderately oxidative. Slow-twitch fibers are fatigue resistant—they are recruited first during a motor act; they generate low tensions; and they usually have a higher capillary/fiber ratio than fast fibers. Fast-twitch fibers can be either fatigue resistant (fast, moderately oxidative) or fast fatiguing (fast glycolytic); they are recruited during motor acts that require large force output. Thus, during normal quiet breathing, it is presumed that only the slow-twitch fibers in the diaphragm are active. In contrast, at the height of an acute attack of croup, asthma, or bronchiolitis during which muscle contractions are strong, both fiber types can be active, with the fast fibers generating the bulk of the force.
Muscle fiber composition, innervation, and metabolism are different in early life. The process of muscle fiber differentiation and interaction with the central nervous system is a continuous process, starting in utero and continuing postnatally. For example, slow oxidative fibers increase in utero and postnatally, whereas fast glycolytic fibers decrease postnatally. Polyneuronal innervation transforms into one motoneuron = one muscle fiber—the adult type of innervation—postnatally. Whether the young infant’s ability to resist muscle fatigue is jeopardized by premature muscle fiber composition, innervation, and metabolism is not known and deserves further investigation.
Respiratory muscle fatigue may arise from central drive fatigue, neural transmission fatigue, contractile fatigue, or a combination of these three phenomena. Many factors predispose respiratory muscles to fatigue. Factors that increase fuel consumption (e.g., increased loads with disease); limit fuel reserves (e.g., malnutrition); alter acid-base homeostasis (e.g., acidosis); modify the oxidative capacity, glycolytic capacity, or both of the muscle (e.g., decreased activity of the muscle and possible atrophy after prolonged artificial ventilation); and decrease the oxygen availability to the muscle (e.g., anemia, low cardiac output states, hypoxemia) all predispose the diaphragm to failure. Reactive oxygen species (free radicals) produced by the contracting diaphragm are also thought to play a role in causing fatigue, particularly in conditions of ischemia/reperfusion and sepsis. In addition, changes in the external milieu of the muscle cell (e.g., low phosphate levels or the presence of certain drugs such as anesthetics) can limit the contractile ability and lead to premature muscle fatigue.
Diaphragmatic muscle function can be assessed clinically by observing the movements of the abdominal wall. During normal inspiration and with the contraction of the diaphragm, the abdominal contents are pushed away from the thorax. Because the abdominal wall is normally compliant during inspiration, the abdominal wall moves out to accommodate the increased pressure from the contracting diaphragm. With diaphragmatic fatigue, weakness, or paralysis, it is possible to observe an inward motion of the abdominal wall. Through the action of other respiratory muscles (intercostals), a drop in pressure occurs in the thorax during inspiration. Because of the “passive” behavior of the fatigued diaphragm, this pressure drop is transmitted to the abdomen; hence, the movement of the abdominal contents toward the thoracic cavity.
The highly compliant chest wall in the newborn infant limits its expansion during inspiration. The chest wall becomes even more unstable during REM sleep, when intercostal muscle activity is inhibited and the rib cage is more prone to distortion. This creates an added load and the potential for diaphragmatic fatigue.
A patient’s respiratory muscle strength can be measured using various techniques, all of which are effort dependent. These include maximum inspiratory and expiratory pressures, sniff pressures, and indirectly by maximal cough flows. If the airway is occluded during normal breathing, the infant, child, and adult diaphragm are all capable of generating airway pressures of greater than 100 cm H 2 O during a maximal inspiratory effort. In the laboratory, respiratory muscle function can be assessed in more detail (e.g., using electromyographic measurements during repeated stimulation of the phrenic nerve). The measurement of transdiaphragmatic pressure (Pdi) by placing balloon catheters just above (esophageal) and below (gastric) the diaphragm can provide a surrogate measure for muscle strength and susceptibility for fatigue, the time tension index (TTI):

P di = mean transdiaphragmatic pressure
P di max = maximal transdiaphragmatic pressure
T i = inspiratory time
T tot = respiratory cycle time
In adults, when the TTI is less than 0.1, it is unlikely that diaphragmatic fatigue will occur.
A less invasive measure involves measuring inspiratory pressures at the mouth instead of across the diaphragm. In this situation,
TTI = ( PI mouth / Pmax mouth ) ∗ ( TI / TTOT )
The caveat for using mouth pressures is that it provides a measurement of force generation during the duty cycle by all respiratory muscles and not just the diaphragm. A TTI > 0.15 has been used to predict unsuccessful extubations in ventilated children.
To consider the main respiratory muscles—the diaphragm and the intercostal muscles—as the only respiratory muscles for breathing is insufficient, especially during stressful conditions or disease states. A number of muscles, such as the alae nasi, pharyngeal wall muscles, genioglossus, posterior cricoarytenoid, and thyroarytenoid, can play major roles in airway patency and hence in ventilatory output. Data indicate that upper airway muscles are strongly recruited during obstructive disease or during inspiratory occlusion, and that blood flow increases considerably to some of them (e.g., genioglossus). How prone these muscles are to fatigue under increased loads is unknown. How different these muscles are in terms of their structure, metabolism, and function in the neonate versus the adult is unclear and needs further research.
Because of the number of muscles involved, their location, and their function, the coordination of respiratory muscles becomes increasingly complex. The motor act of respiration should no longer be viewed as the result of one or two muscles contracting during inspiration and relaxing during expiration. At rest and even more so during disease states, the active coordination of various muscles becomes functionally very important. Defecation, sucking, and talking all involve the activation of several muscles that are shared by the respiratory apparatus for generating adequate ventilation. In some cases, obstructive apneas can actually be the result of muscle incoordination, with the diaphragm contracting when upper airway muscles that normally hold the airway open are relaxed.
Gas Exchange
The vital process of gas exchange occurs in the terminal respiratory unit. The previous sections of this chapter deal with the problems of moving air and blood to and from these gas-exchanging units. This section focuses on the fate of gas once it is introduced into the lungs, how it is transferred from the alveolar space to the bloodstream, and how ventilation and perfusion are matched.
In Fig. 6.23 , the partial pressures of oxygen and carbon dioxide are depicted at various stages of the pathway from ambient air to the tissues. Because nitrogen is inert, changes in its partial pressure (P n 2 ) in the gas phase depend on changes in the partial pressures of oxygen and carbon dioxide, gases that are utilized and excreted, respectively. In contrast, P n 2 in blood and tissue is identical because nitrogen is inert. The rather complex influences of dead space, alveolar ventilation, ventilation-perfusion relationships, and tissue metabolism on the partial pressures of oxygen and carbon dioxide are discussed in some detail, and frequent reference to Fig. 6.23 is useful in clarifying some of the concepts.

Alveolar Ventilation
One component of the V t is the anatomic dead space, V d (consisting of the nose, mouth, pharynx, larynx, trachea, bronchi, and bronchioles), where no significant exchange of oxygen and carbon dioxide with blood takes place. The other component of the V t , V a , undergoes gas exchange in the alveoli. Alveolar ventilation per minute is measured by the following equation:
VA = VT − VD
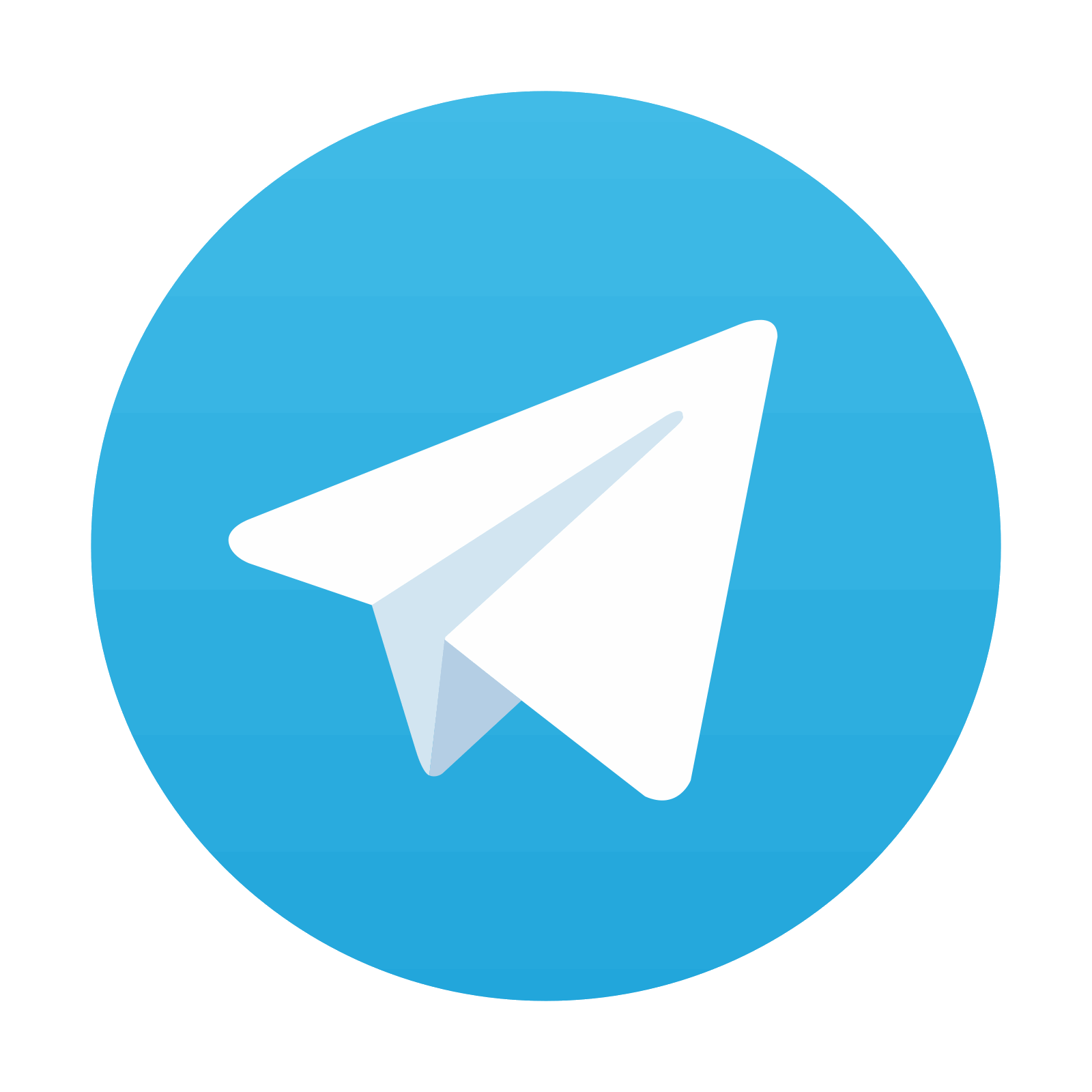
Stay updated, free articles. Join our Telegram channel
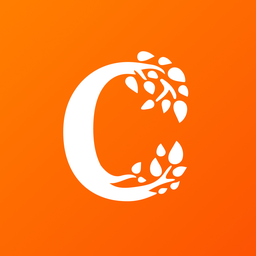
Full access? Get Clinical Tree
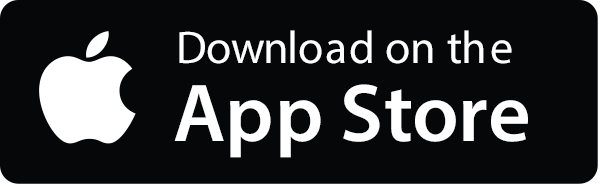
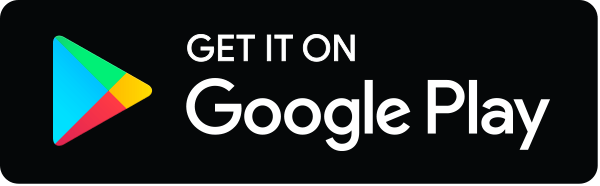