Chapter 54 The Liver
Anatomy and Physiology
Anatomy
Gross Anatomy
General Description and Topography
On the posterior surface of the left liver, running from the left portal vein in the porta hepatis toward the left hepatic vein and the IVC, is the ligamentum venosum (obliterated sinus venosus) which also runs in a fissure (Fig. 54-1). Hepatic arterial and portal venous blood enter the liver at the hilum and branch throughout the liver as a single portal pedicle unit, which also includes a bile duct. These portal triads are invested in a peritoneal sheath that invaginates at the hepatic hilum. Venous drainage is through the right, middle, and left hepatic veins that empty directly into the suprahepatic IVC.
Normal Development and Embryology
The developing liver shares a common progenitor with the biliary tree and pancreas. During embryogenesis, signals are transmitted from the cardiac mesenchyme and septum transversum. The molecules regulating this (e.g., FGF, BMP, Wnt) have begun to be elucidated. The liver primordium begins to form in the third week of development as an outgrowth of endodermal epithelium, known as the hepatic diverticulum or liver bud, known as the hepatic field. The connection between the hepatic diverticulum and the future duodenum narrows to form the bile duct and an outpouching of the bile duct forms into the gallbladder and cystic duct. Hepatic cells develop cords and intermingle with the vitelline and umbilical veins to form hepatic sinusoids. Simultaneously, hematopoietic cells, Kupffer cells and connective tissue form from the mesoderm of the septum transversum. The mesoderm of the septum transversum connects the liver to the ventral abdominal wall and foregut. As the liver protrudes into the abdominal cavity, these structures are stretched into thin membranes, ultimately forming the falciform ligament and lesser omentum, respectively. The mesoderm on the surface of the developing liver differentiates into visceral peritoneum, except superiorly, where contact between the liver and mesoderm (future diaphragm) is maintained, forming a bare area devoid of visceral peritoneum (Fig. 54-2).
The primitive liver plays a central role in the fetal circulation. The vitelline veins carry blood from the yolk sac to the sinus venosus and ultimately form a network of veins around the foregut (future duodenum) that drain into the developing hepatic sinusoids. These vitelline veins eventually fuse to form the portal, superior mesenteric, and splenic veins. The sinus venosus, which empties into the fetal heart, becomes the hepatocardiac channel and then the hepatic veins and retrohepatic IVC. The umbilical veins, which are paired early on, carry oxygenated blood to the fetus. Initially, the umbilical veins drain into the sinus venosus but at week 5 of development, they begin to drain into the hepatic sinusoids. The right umbilical vein ultimately disappears and the left umbilical vein later drains directly into the hepatocardiac channel, bypassing the hepatic sinusoids through the ductus venosus. In the adult liver, the remnant of the left umbilical vein becomes the ligamentum teres, which runs in the falciform ligament into the umbilical fissure, and the remnant of the ductus venosus becomes the ligamentum venosus at the termination of the lesser omentum under the left liver (Fig. 54-3).
Functional Anatomy
The functional anatomy of the liver (Figs. 54-4 and 54-5) is composed of eight segments, each supplied by a single portal triad (also called a pedicle) composed of a portal vein, hepatic artery, and bile duct. These segments are further organized into four sectors separated by scissurae containing the three main hepatic veins. The four sectors are even further organized into the right and left liver. The terms right liver and left liver are preferable to the terms right lobe and left lobe because there is no external mark that allows the identification of the right and left liver. This system was originally described in 1957 by Woodsmith and Goldburne, and by Couinaud. It defines hepatic anatomy because it is most relevant to surgery of the liver. The functional anatomy is more often seen as cross-sectional imaging (Fig. 54-6).
At the hilum of the liver, the right portal triad has a short extrahepatic course of approximately 1 to 1.5 cm before entering the substance of the liver and branching into anterior and posterior sectoral branches. The left portal triad, however, has a long extrahepatic course of up to 3 to 4 cm and runs transversely along the base of segment 4 in a peritoneal sheath, which is the upper end of the lesser omentum. This connective tissue is known as the hilar plate (Fig. 54-7). The continuation of the left portal triad runs anteriorly and caudally in the umbilical fissure and gives branches to segments 2 and 3 and recurrent branches to segment 4.
The caudate lobe (segment 1) is the dorsal portion of the liver. It embraces the IVC on its posterior surface and lies posterior to the left portal triad inferiorly and the left and middle hepatic veins superiorly. The main bulk of the caudate lobe is to the left of the IVC, but inferiorly it traverses between the IVC and left portal triad, where it fuses to the right liver (segments 6 and 7). This part of the caudate lobe is known as the right portion or the caudate process. The left portion of the caudate lobe lies in the lesser omental bursa and is covered anteriorly by the gastrohepatic ligament (lesser omentum) that separates it from segments 2 and 3 anteriorly. The gastrohepatic ligament attaches to the ligamentum venosum (sinus venosus remnant) along the left side of the left portal triad (Fig. 54-8).
Portal Vein
The portal vein divides into main right and left branches at the hilum of the liver. The left branch of the portal vein runs transversely along the base of segment 4 and into the umbilical fissure, where it gives off branches to segments 2 and 3 and feedback branches to segment 4. The left portal vein also gives off posterior branches to the left side of the caudate lobe. The right portal vein has a short extrahepatic course; it usually enters the substance of the liver where it splits into anterior and posterior sectoral branches. These sectoral branches can occasionally be seen extrahepatically and can come off the main portal vein before its bifurcation. There is usually a small caudate process branch off the main right portal vein or at the right portal vein bifurcation, which comes off posteriorly to supply this portion of liver (Fig. 54-9).
Hepatic Artery
The common description of the arterial supply to the liver and biliary tree is only present approximately 60% of the time (Fig. 54-10). The celiac trunk originates directly off the aorta, just below the aortic diaphragmatic hiatus, and gives off three branches—the splenic artery, left gastric artery, and common hepatic artery. The common hepatic artery passes forward and to the right along the superior border of the pancreas and runs along the right side of the lesser omentum, where it ascends towards the hepatic hilum, lying anterior to the portal vein and to the left of the bile duct. At the point where the common hepatic artery begins to head superiorly towards the hepatic hilum, it gives off the gastroduodenal artery, followed by the supraduodenal artery and right gastric artery. The common hepatic artery beyond the takeoff of the gastroduodenal is called the proper hepatic artery; it divides into right and left hepatic arteries at the hilum. The left hepatic artery heads vertically towards the umbilical fissure to supply segments 2, 3, and 4. The left hepatic artery usually also gives off a middle hepatic artery branch that heads toward the right side of the umbilical fissure and supplies segment 4. The right hepatic artery usually runs posterior to the common hepatic bile duct and enters Calot’s triangle, bordered by the cystic duct, common hepatic duct, and liver edge, where it gives off the cystic artery to supply the gallbladder and then continues into the substance of the right liver.
Unlike portal vein anatomy, hepatic arterial anatomy is extraordinarily variable (Fig. 54-11). An accessory vessel is described as an aberrant origin of a branch that is in addition to the normal branching pattern. A replaced vessel is described as an aberrant origin of a branch that substitutes for the lack of the normal branch. Usually, the hepatic artery originates off the celiac trunk. However, branches or the entire hepatic arterial system can originate off the superior mesenteric artery (SMA). The right and left hepatic arteries can also arise separately off the celiac axis. Replaced or accessory right hepatic arteries come off the SMA and are present approximately 11% to 21% of the time. Hepatic vessels replaced to the SMA run behind the head of the pancreas, posterior to the portal vein in the portacaval space. The right hepatic artery, in its usual branching pattern, can also course anterior to the common hepatic duct. A replaced or accessory left hepatic artery is present approximately 3.8% to 10% of the time, originates from the left gastric artery, and courses within the lesser omentum, heading toward the umbilical fissure. Other important variations include the origin of the gastroduodenal artery, which has been found to originate from the right hepatic artery and is occasionally duplicated. The anatomy of the cystic artery is also variable; knowledge of these variations is of particular importance in the performance of cholecystectomy (Fig. 54-12). An accessory cystic artery can originate from the proper hepatic artery or gastroduodenal artery, where it runs anterior to the bile duct. A single cystic artery can originate anywhere off the proper hepatic artery or gastroduodenal artery, or directly from the celiac axis. These variant cystic arteries can run anterior to the bile duct and are not necessarily present in the triangle of Calot. All these variations in hepatic arterial anatomy are of obvious importance during hepatic resection, hepatic arterial pump placement, cholecystectomy, and hepatic interventional radiologic procedures.
Hepatic Veins
The three major hepatic veins drain from the superior-posterior surface of the liver directly into the IVC (see Figs. 54-4, 54-5, and 54-6). The right hepatic vein runs in the right scissura between the anterior and posterior sectors of the right liver and drains most of the right liver after a short (1-cm) extrahepatic course into the right side of the IVC. The left and middle hepatic veins usually join intrahepatically and enter the left side of the IVC as a single vessel, although they may drain separately. The left hepatic vein runs in the left scissura between segments 2 and 3 and drains segments 2 and 3; the middle hepatic vein runs in the portal scissura between segment 4 and the anterior sector of the right liver, comprised of segments 5 and 8, and drains segment 4 and some of the anterior sector of the right liver. The umbilical vein is an additional vein that runs under the falciform ligament, between the left and middle veins, and usually empties into the left hepatic vein. A number of small posterior venous branches from the right posterior sector and caudate lobe drain directly into the IVC. A substantial inferiorly located accessory right hepatic vein is commonly encountered. There is also often a venous tributary from the caudate lobe, which drains superiorly into the left hepatic vein.
Biliary System
The gallbladder is a biliary reservoir that lies against the inferior surface of segments 4 and 5 of the liver, usually making an impression against the liver. A peritoneal layer covers most of the gallbladder, except for the portion adherent to the liver. Here, the gallbladder adheres to the liver by a layer of fibroconnective tissue known as the cystic plate, an extension of the hilar plate (see Fig. 54-7). Variable in size, but usually about 10 cm long and 3 to 5 cm wide, the gallbladder is composed of a fundus, body, infundibulum, and neck, which ultimately empty into the cystic duct. The fundus usually projects just slightly beyond the liver edge anteriorly and, when folded on itself, is described as a Phrygian cap. Continuing toward the bile duct, the body of the gallbladder is usually in close proximity to the second portion of the duodenum and transverse colon. The infundibulum (or Hartmann’s pouch) hangs forward along the free edge of the lesser omentum and can fold in front of the cystic duct. The portion of gallbladder between the infundibulum and cystic duct is referred to as the neck. The cystic duct is variable in its length, course, and insertion into the main biliary tree. The first portion of the cystic duct is usually tortuous and contains mucosal duplications, referred to as the folds of Heister, which regulate the filling and emptying of the gallbladder. Usually, the cystic duct joins the common hepatic duct to form the common bile duct.
Knowledge of the multiple and frequent variations in the anatomy of the biliary tree is absolutely essential for performing hepatobiliary procedures. Anomalies of the hepatic ductal confluence are common and are present approximately one third of the time. The most common anomalies of the biliary confluence involve variations in the insertion of the right sectoral ducts. Usually, this is the posterior sectoral duct. The confluence can be a trifurcation of the right anterior sectoral, right posterior sectoral, and left hepatic ducts. Either of the right sectoral ducts can drain into the left hepatic duct, common hepatic duct, cystic duct or, rarely, the gallbladder (Fig. 54-13).
The position and entry of the cystic duct into the main ductal system are also variable. Double cystic ducts draining a unilocular gallbladder and drainage into hepatic duct branches have been reported. Usually, the cystic duct joins the common hepatic duct at an angle, but can run parallel and enter it more distally. In the latter situation, the cystic duct can be fused to the hepatic duct along its parallel course by connective tissue. The cystic duct can also run a spiral course anteriorly or posteriorly and enter the left side of the common hepatic duct. Finally, the cystic duct can be very short or even absent (Fig. 54-14).
The supraduodenal and infrahilar bile duct are predominantly supplied by two axial vessels that run at 3- and 9-o’clock positions. These vessels are derived from the superior pancreaticoduodenal, right hepatic, cystic, gastroduodenal, and retroduodenal arteries. It has been estimated that only 2% of the arterial supply to this portion of the bile duct is segmental, arising directly off the proper hepatic artery. The bile duct and its bifurcation in the hilum derive their arterial blood supply from a rich network of multiple small branches from surrounding vessels. Similarly, the retropancreatic bile duct derives its arterial supply from the retroduodenal artery, which provides a rich network of multiple small branches (Fig. 54-15). Venous drainage of the bile duct parallels the arterial supply and drains into the portal venous system. The venous drainage of the gallbladder empties into the veins that drain the bile duct and does not flow directly into the portal vein.
Microscopic Anatomy
Functional Unit of the Liver
The organization of hepatic parenchyma into microscopic functional units has been described in a number of ways, referred to as an acinus or a lobule (Fig. 54-16). This was originally described by Rappaport and then modified by Matsumoto and Kawakami.6 A lobule is made up of a central terminal hepatic venule surrounded by four to six terminal portal triads that form a polygonal unit. This unit is lined on its periphery between each terminal portal triad by terminal portal triad branches. In between the terminal portal triads and the central hepatic venule, hepatocytes are arranged in one cell–thick plates, surrounded on each side by endothelial-lined and blood-filled sinusoids. Blood flows from the terminal portal triad through the sinusoids into the terminal hepatic venule. Bile is formed within the hepatocytes and empties into terminal canaliculi, which form on the lateral walls of the intercellular hepatocyte. These ultimately coalesce into bile ducts and flow toward the portal triads. This functional hepatic unit provides a structural basis for the many metabolic and secretory functions of the liver.
Hepatic Microcirculation
The endothelium-lined sinusoids of the hepatic lobule represent the functional unit of the liver, where afferent blood flow is exposed to functional hepatic parenchyma prior to being drained into hepatic venules (Fig. 54-17). The hepatic sinusoids are 7 to 15 µm wide but can increase in size by up to 10-fold. This yields a low-resistance and low-pressure (generally, 2 to 3 mm Hg) system. The sinusoidal endothelial cells account for 15% to 20% of the total hepatic cell mass.
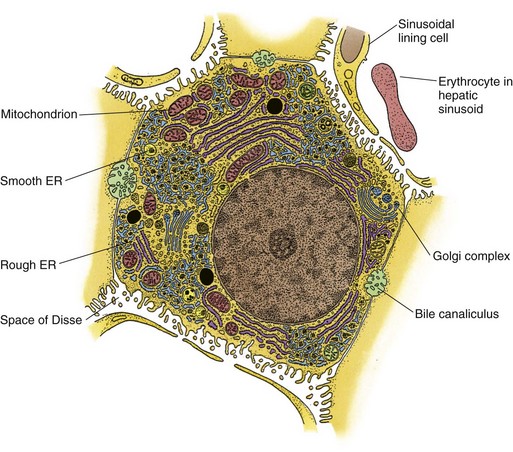
FIGURE 54-17 A hepatocyte and its sinusoidal and lateral domains. ER, endoplasmic reticulum.
(From Ross MH, Reith EJ, Romrell LJ. The liver. In Ross RH, Reith EJ, Romrell LJ: Histology: A Text and Atlas. Baltimore, Williams & Wilkins; 1989, pp 471–478.)
Hepatocytes
Hepatocytes are complex multifunctional cells that make up 60% of the hepatic cellular mass and 80% of the cytoplasmic mass of the liver (see Fig. 54-17). Morphologically, the hepatocyte is a polyhedral cell with a central spherical nucleus. As noted, hepatocytes are arranged in single cell layer plates lined on either side by blood-filled sinusoids. Every hepatocyte has contact with adjacent hepatocytes, the biliary space (bile canaliculus), and the perisinusoidal space, enabling these cells to perform their broad range of functions. Among the many essential functions of the hepatocyte are the following: (1) uptake, storage and release of nutrients; (2) synthesis of glucose, fatty acids, lipids, and numerous plasma protein (including C-reactive protein and albumin); (3) production and secretion of bile for digestion of dietary fats; and (4) degradation and detoxification of toxins.
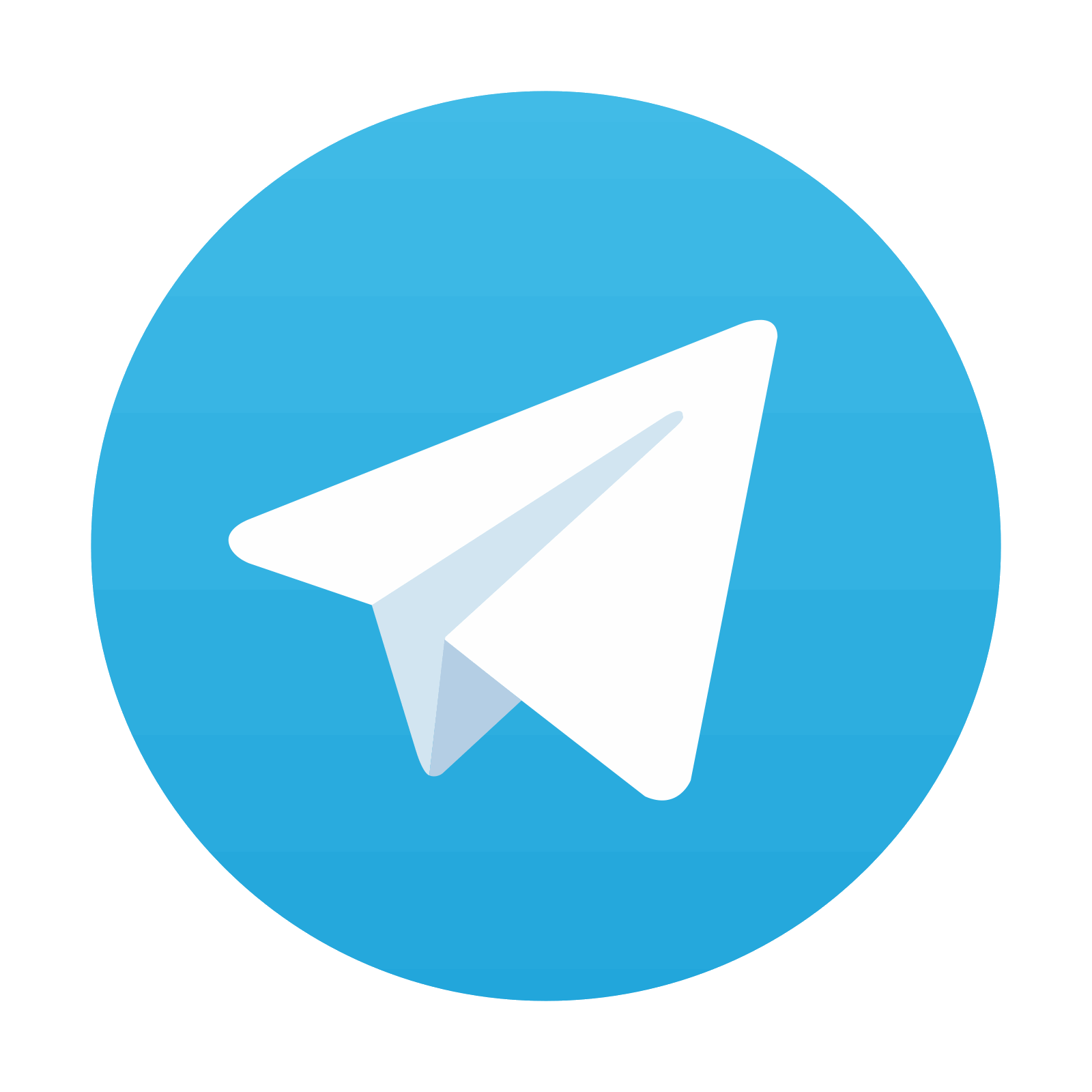
Stay updated, free articles. Join our Telegram channel
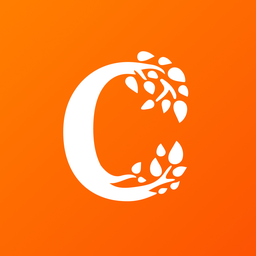
Full access? Get Clinical Tree
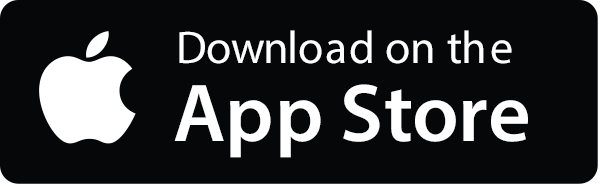
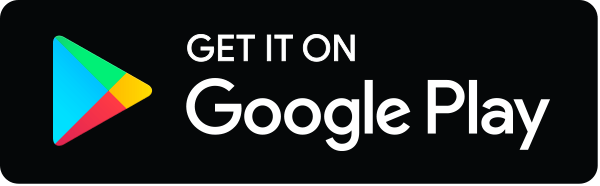