Background
The aims of this study were to assess the influence of left bundle branch block (LBBB)–like conduction abnormalities on left ventricular (LV) blood flow patterns and to characterize their potential impact on LV efficiency by measuring the changes in vortex formation and energy dissipation in the left ventricle using echocardiographic particle image velocimetry.
Methods
Thirty-six subjects were prospectively studied, including 20 patients with pacemakers, six patients with LBBB, and 10 healthy control subjects, all of whom had normal ejection fractions (>50%). In patients with pacemakers, data were acquired in both DDD and AAI modes. Standard grayscale, tissue Doppler myocardial imaging, and contrast-enhanced echocardiographic particle image velocimetric data were acquired, and LV flow patterns were analyzed using dedicated software. Dyssynchrony was quantified by measuring apical transverse motion.
Results
Apical transverse motion was significantly higher in patients with LBBB compared with normal control subjects (mean, 4.9 ± 1.9 vs 1.0 ± 0.7 mm; P < .001). Quantitative measures of vortex energy dissipation (relative strength, vortex relative strength, and vortex pulsation correlation) were significantly higher in patients with LBBB (2.05 ± 0.54, 0.53 ± 0.13, and 0.87 ± 0.47, respectively) compared with control subjects (1.48 ± 0.28, 0.33 ± 0.05, and 0.24 ± 0.51, respectively) ( P < .02 for all). Vortex duration time in relation to the entire cardiac cycle was shorter in patients with LBBB than in control subjects (28% vs 44%). All findings in both groups were comparable with DDD and AAI.
Conclusion
LV flow pattern analysis by echocardiographic particle image velocimetry reveals that conduction delay due to LBBB or pacemaker stimulation in the right ventricle (DDD) disturbs the transfer of kinetic energy during the cardiac cycle and causes less efficient LV function. These data contribute to a better understanding of hemodynamic consequences of conduction delays and may help in the optimization of therapeutic approaches.
Vortices are complex flow structures that contain kinetic energy due to rotating fluid. In two dimensions, vortical flow can be quantified by the parameter vorticity (ω), which describes the difference of the gradient of the y component of the flow velocity in the x direction (∂ V y ∂ x ) and the gradient of the x component of the flow velocity in the y direction (∂ V x ∂ y ) according to the following formula:
Accordingly, a counterclockwise vortex has positive vorticity and a clockwise vortex has negative vorticity, while laminar flow has zero vorticity.
Vortices occur when laminar flow detaches from a sharp edge. In the human left ventricle, diastolic filling triggers vortex formation at the tips of the mitral valve leaflets. As the moving blood of a vortex stores kinetic energy, it thereby facilitates its transmission from diastolic inflow to systolic outflow and reduces the need for reacceleration of the blood before ejection, which increases left ventricular (LV) efficiency. Any pathology that disturbs the normal interplay of chamber mechanics and hemodynamics by preventing vortex formation might therefore impair ventricular efficiency.
Although a singular, large vortex is a relatively steady structure, turbulence is characterized by many fast changing vortices that cause a rapid dissipation of kinetic energy. The amount of regional change in vortex structures has been referred to as “pulsatility” and can be described for the entire left ventricle as relative pulsatile vorticity strength or, for the vortex only, vortex relative pulsatile vorticity strength and the correlation between steady and pulsatile vorticity. All three parameters have been proposed as indicators of energy dissipation in the human left ventricle.
Particle image velocimetry (PIV) is an image analysis method that enables the determination of flow patterns by tracking particles that move together with the fluid. Recently, this method has been successfully applied to contrast enhanced echocardiographic images. Initial studies showed that contrast tracking delivers sufficiently accurate information for the detection of vortex structures and the calculation of vorticity and other derived parameters.
Electrical conduction abnormalities such as left bundle branch block (LBBB) patterns are common in patients with heart failure. Similarly, therapeutic right ventricular apical pacing can induce conduction abnormalities comparable with LBBB and lead to systolic dyssynchrony. In both cases, the sequentially activated LV myocardial regions prestretch each other, which results in inefficient ventricular work. Consequently, hearts with LBBB-like conduction delays generate less stroke volume, have prolonged isovolumetric time intervals, and have disturbed filling. It is unknown, however, to what extent the abnormal sequence of LV wall contraction interacts with vortex formation and the transfer of kinetic energy from diastole to systole.
The aims of this study were therefore to investigate the influence of LBBB-like conduction abnormalities on LV blood flow patterns and to characterize their potential impact on LV efficiency by measuring the changes in vortex formation and energy dissipation in the left ventricle by means of echocardiographic PIV.
Methods
Study Population
A total of 54 subjects were prospectively screened for this study, including 23 patients with pacemakers, 21 patients with LBBB, and 10 volunteers. Bad echogenicity, reduced LV ejection fraction (<50%), regional dysfunction, more than mild valvular disease, pulmonary hypertension, previous myocardial infarction, percutaneous coronary intervention, cardiac surgery, atrial or ventricular arrhythmia, and any unstable cardiovascular condition were exclusion criteria.
Pacemaker Group
This group was recruited from the pacemaker clinic of our department (University Hospital Gasthuisberg, Leuven, Belgium). All patients underwent pacemaker testing and screening echocardiography before inclusion. We selected patients with dual-chamber pacemakers capable of working in AAI and DDD modes, with the ventricular lead placed at the right ventricular apex. Care was taken that all atrioventricular or ventricular conduction delays were intermittent and not present at the time of the investigation. Patients with permanent atrioventricular block, any intrinsic bundle branch block, or inadequate echocardiographic image quality were excluded. From 23 screened patients, three were excluded because of bad echocardiographic image quality.
LBBB Group
Patients were recruited by screening electrocardiograms from the electrocardiography service of our department. As in pacemaker patients, all patients with LBBB had undergone screening echocardiography before inclusion to rule out structural heart disease and to check for adequate echocardiographic image quality. Six patients with idiopathic LBBB and normal global LV function were included. All were in sinus rhythm, without any atrial or ventricular arrhythmia. The absence of coronary artery disease was confirmed by coronary angiography in all patients. Among 21 screened patients, 15 had to be excluded because of LV dysfunction ( n = 3), coronary artery disease ( n = 3), atrial fibrillation ( n = 5), and bad image quality ( n = 4).
Normal Control Subjects
Finally, 10 healthy volunteers without any histories of cardiovascular disease and with normal findings on electrocardiography, physical examination, and echocardiography were recruited to participate in this study. All screened volunteers could be included.
All participants gave written informed consent before inclusion. The study was approved by the local ethics committee.
Pacing Protocol
Pacemakers were programmed to a heart rate slightly higher than the intrinsic sinus activity to allow constant atrial pacing. All echocardiographic data were acquired twice, in both AAI and DDD pacing modes. Five minutes of hemodynamic adaptation were allowed after mode change. The order of modes was random. For DDD pacing, the atrioventricular delay was adjusted so that full ventricular capture was achieved, but no A-wave truncation occurred.
Echocardiographic Image Acquisition
A commercially available Vivid 7 ultrasound scanner was used (GE Vingmed Ultrasound AS, Horten, Norway) to acquire complete standard transthoracic echocardiograms. To characterize regional myocardial function, color tissue Doppler myocardial imaging data were acquired from parasternal (parasternal long-axis) and apical (four-, three-, and two-chamber view) windows with optimized sector and depth settings to achieve high frame rates.
For echocardiographic PIV, dedicated two-dimensional grayscale images with the highest possible frame rate (95–110 frames/sec) were acquired from three apical planes using a Siemens Acuson Sequoia ultrasound system (Siemens Medical Solutions USA, Mountain View, CA). We used SonoVue (Bracco, Milan, Italy) as a contrast agent, which contains microbubbles in a concentration of 1 × 10 8 to 5 × 10 8 per milliliter. This suspension was injected intravenously as a bolus (0.1–0.2 mL) followed by 10 mL of saline flush. Because a high-contrast bubble concentration causes shadowing in the deeper parts of the image, we carefully waited for a sufficient decay of the bubble concentration in the LV cavity, so that single bubbles could be clearly distinguished from one another in the entire chamber. Only then were contrast bubbles imaged, with a mechanical index of 0.4 to 0.5 to minimize further bubble destruction. From each acquisition, three consecutive heart cycles were stored for later postprocessing. All participants underwent the same scanning protocol, pacemaker patients twice, in both AAI and DDD modes.
Postprocessing and Measurements
Standard Imaging
An EchoPAC workstation (BT 09; GE Vingmed Ultrasound AS) was used to measure cardiac diameters, volumes, ejection fraction (using the biplane Simpson method), conventional Doppler parameters, and valve timing data. From the latter, phases of the cardiac cycle were defined. Diastole was divided into early diastole, diastasis, and late diastole. Diastasis was defined as the period between end of the E wave and onset of the A wave in the mitral inflow signal ( Figure 1 ). Ejection fraction estimates were averaged from independent readings of two experienced observers.

Echocardiographic PIV
Two-dimensional grayscale contrast images of three apical planes were analyzed offline using dedicated software (Q Flow version 2.4.6; Siemens Healthcare, Erlangen, Germany). After the definition of QRS onset, endocardial borders were delineated manually and the LV cavity was automatically tracked by the software. Afterward, the intracavitary region was processed, obtaining detailed intraventricular flow tracking data. Quantitative parameters of vorticity (ω), the average main vortex position and size (vortex depth [VD] and vortex length [VL]) as well as energy dissipation (relative strength [RS], vortex RS [VRS], and vortex pulsation correlation [VPC]) were automatically derived by the software, displayed in color-coded images ( Figure 2 ). Red color indicated a counterclockwise vortex, which is the main vortex as visualized in an apical four-chamber view, while blue color indicated clockwise vortex flow, which is normally observed in the apical long-axis and apical two-chamber views. Because the behavior of quantitative vortex parameters was comparable in all scan planes, only results of the four-chamber view are reported. Lower VD values indicate a more basal position of the average main vortex relative to the LV longitudinal axis. The area of the main vortex in relation to LV cavity area was assessed throughout the cardiac cycle as VA rel ( Figure 2 ). The vortex duration time was defined as the time between the onset of vortex formation and its disappearance and expressed in relation to the length of the entire cardiac cycle and of the different time intervals.

Wall Motion Analysis by Tissue Doppler
Myocardial velocity and strain rate traces were extracted from the tissue Doppler data by placing regions of interest at the basal, medial, and apical segments of each myocardial wall (septal, lateral, anteroseptal, posterior, anterior, and inferior). An additional, far apical velocity trace was extracted from each wall for the later calculation of apical transverse motion (ATM) as a quantitative description of apical rocking ( Figure 3 ). All region-of-interest positions were manually tracked during the cardiac cycle to follow myocardial motion. Traces were stored numerically for further processing.

Dedicated MATLAB-based (The MathWorks, Natick, MA) analysis software (TVA version 14.7; J.-U. Voigt, Leuven, Belgium) was used for the processing and analysis of the tissue Doppler–derived velocity and strain rate traces. In strain curves, we measured strain during ejection time (ε et ) and strain during the entire cardiac cycle (ε tot ). The ratio ε et /ε tot , averaged over all 18 myocardial segments, describes the fraction of myocardial contraction that contributes to ejection and was therefore used as indicator of LV contraction efficiency. Furthermore, the difference in average shortening during ejection time in the septal and lateral wall (ε diff_4CV = ε et[lat] − ε et[sep] ) was calculated. Typical strain patterns are shown in Figure 4 .

ATM
In a heart with LBBB-like motion, the typical excursion pattern of the apex is commonly referred to as “apical rocking” and considered a sign of mechanical dyssynchrony. ATM quantifies apical rocking by measuring the apical excursion perpendicular to the LV long axis. It was calculated from the far apical color tissue Doppler velocity traces, as previously described. In short, the integral of the velocity curves (i.e. the longitudinal motion curves) of the far apical regions of interest are averaged while inverting the right-sided curve of the image. Assuming that the apex is a homogeneous “cap,” the result can be interpreted as reflecting ATM. Values were expressed in millimeters.
Statistical Analysis
All continuous variables are expressed as mean ± SD. For multiple-group comparisons, analysis of variance was used with Bonferroni correction for post hoc pairwise comparisons. The Pearson coefficient ( r ) was used to assess correlations. To evaluate the ability of ATM 4CV to distinguish between normal and LBBB-like conduction, a receiver operating characteristic curve was constructed and area under the curve was calculated. Ten randomly selected data sets were reanalyzed to determine the reproducibility of strain, ATM measurements, and postprocessed vortex parameters by means of a Bland-Altman analysis. We further calculated the intraclass correlation coefficient (with 95% CI) and the coefficient of variation between two readings. All statistical calculations were performed using SPSS version 16.0 (SPSS, Chicago, IL). P values < .05 were considered to indicate statistical significance.
Results
Study Population, Hemodynamic, and Echocardiographic Characteristics
Table 1 presents the characteristics of the study population. Heart rate was not significantly different between groups and was identical between AAI and DDD pacing modes. QRS was significantly wider in the DDD and LBBB groups than in the AAI and normal control groups ( P < .001 for both). Analysis of variance showed no significant differences in volumes, diameters, and mitral inflow velocities of the ventricles in the different groups, while it revealed a minor but significant decrease of ejection fraction in the pacemaker patients when paced in DDD mode instead of AAI mode ( P < .02).
Variable | Normal control subjects ( n = 10) | P ∗ | AAI pacing ( n = 20) | P † | DDD pacing ( n = 20) | P ‡ | Patients with LBBB ( n = 6) | P § |
---|---|---|---|---|---|---|---|---|
Age (y) | 51 ± 9 | 67 ± 13 | 67 ± 13 | 55 ± 14 | ||||
Heart rate (beats/min) | 65 ± 10 | 68 ± 6 | 68 ± 6 | 66 ± 9 | ||||
QRS duration (msec) | 100 ± 7 | 90 ± 12 | .00 | 191 ± 34 | .00 | 163 ± 17 | .00 | |
LV EF (%) | 64 ± 5 | 64 ± 3 | .02 | 61 ± 3 | 60 ± 5 | |||
LV ESV (mL) | 22 ± 7 | 24 ± 5 | 27 ± 6 | 27 ± 7 | ||||
LV EDV (mL) | 61 ± 17 | 68 ± 13 | 70 ± 14 | 67 ± 16 | ||||
LV ESD (mm) | 24 ± 2 | 25 ± 3 | 27 ± 3 | 26 ± 3 | ||||
LV EDD (mm) | 36 ± 3 | 38 ± 4 | 40 ± 4 | 38 ± 5 | ||||
LAD (mm) | 28 ± 3 | 34 ± 3 | 32 ± 7 | 33 ± 3 | ||||
Aortic VTI | 26 ± 5 | 31 ± 6 | 27 ± 5 | 34 ± 15 | ||||
E velocity (m/sec) | 0.76 ± 0.1 | 0.65 ± 0.1 | 0.63 ± 0.1 | 0.85 ± 0.4 | ||||
A velocity (m/sec) | 0.71 ± 0.1 | 0.80 ± 0.2 | 0.79 ± 0.2 | 0.81 ± 0.1 | ||||
E/A ratio | 1.14 ± 0.4 | 0.87 ± 0.4 | 0.90 ± 0.3 | 1.01 ± 0.2 |
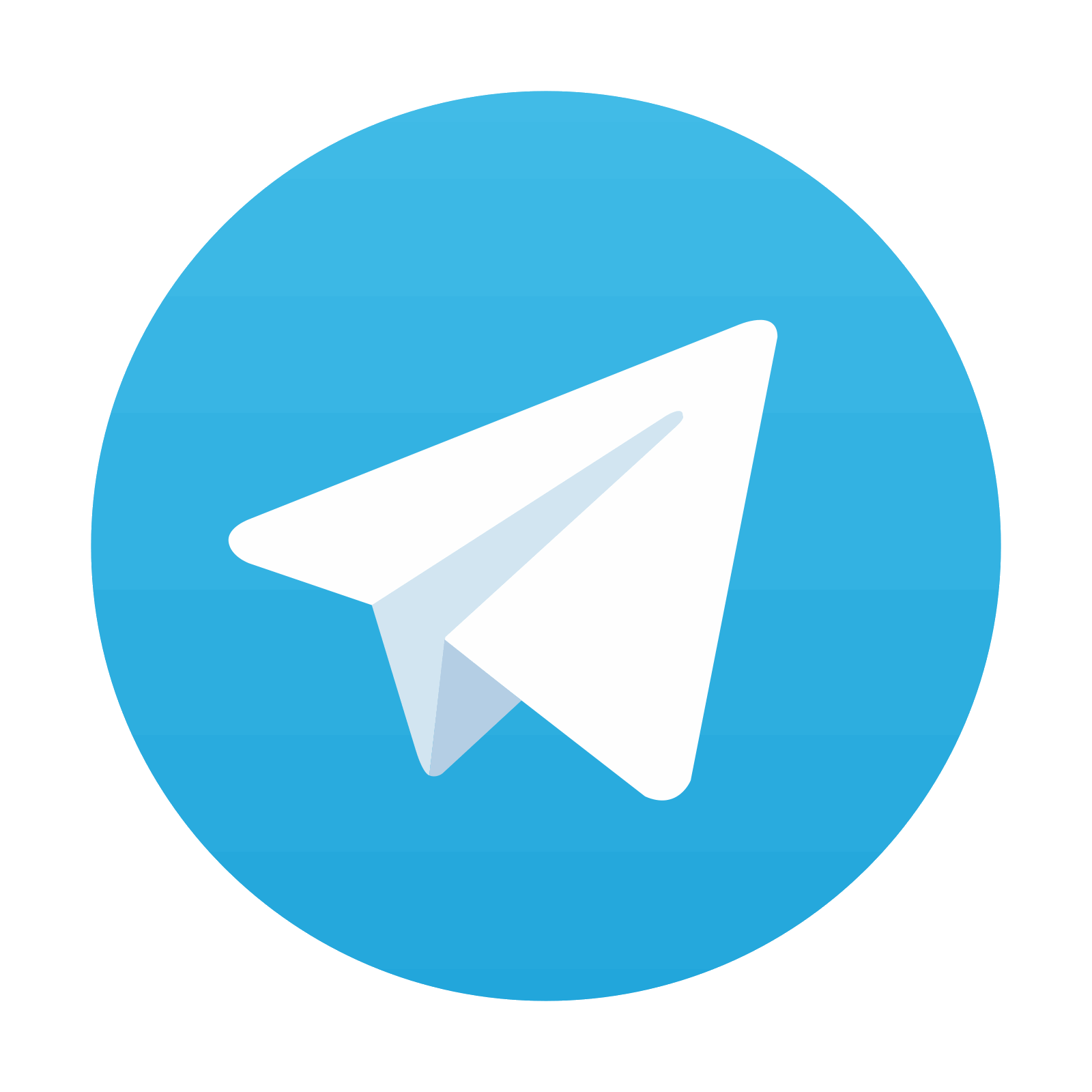
Stay updated, free articles. Join our Telegram channel
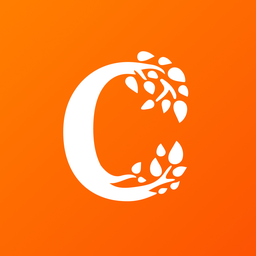
Full access? Get Clinical Tree
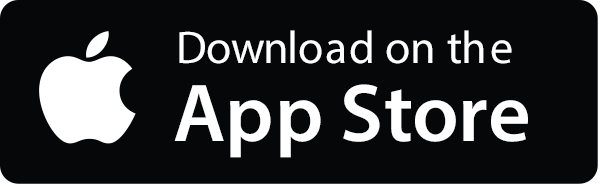
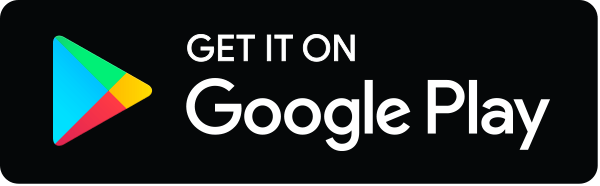
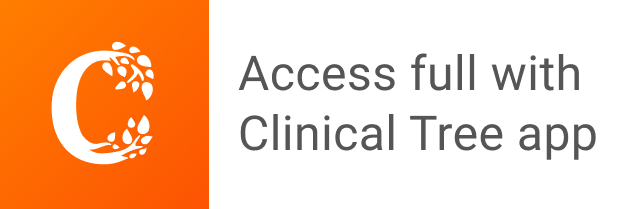