Each and every blood vessel throughout the body is covered, at its inner, luminal surface, by a monolayer of specialized cells, i.e., the vascular endothelial cells. This monolayer represents the primary anatomical site that separates the compartment of the flowing blood from the body’s interstitium. Although spread throughout the body and thus not easily discernible, all endothelial cells together cover a surface that has been estimated as 350 m2 (see Ref. 1) and may count as many as 60 trillion (6 × 1013) cells.2 The total weight of all endothelial cells has been estimated to be between 110 g3 and 750 g, or as much as the liver.4 Recent scientific evidence has made clear that the endothelium may not be less metabolically active than the liver. It was 25 years ago when the ground-breaking experimental studies discovered the crucial role of the endothelium in regulating vascular smooth muscle tone and coagulation and it was concluded that the endothelium is not merely a passive barrier.5,6,7
Endothelial cells are polygonal in shape and generally orientated along the long axis of the vessels, thereby responding to the forces that the shear of the flowing blood exerts on their surface. Endothelial cells are polarized cells, with an apical (luminal) membrane facing the blood stream and an abluminal membrane facing the intercellular space. This polarity is manifested by a distinct protein composition of the two membranes and the controlled transport of molecules along either of these membranes. Intercellular tight junctions impede intercellular diffusion of molecules between the apical and abluminal membrane.
Because of its position, the endothelium is permanently exposed to hemodynamic forces exerted by blood flow, blood pressure, and vascular wall distension. In addition to these mechanical stimuli, the endothelium receives a plethora of chemical signals, both blood-borne and tissue-derived, which may elicit endothelial responses acting on the vessel wall itself or on more distant target sites. Some of these signals play an important role in modifying the primary function of the endothelium, that is, the precise control of the passage of solutes, macromolecules, and blood cells across the vascular wall.
Morphologically, endothelial cells are flat cells that usually spread out broadly on the inner surface of a blood vessel; the nucleus can be prominently seen on microscopic images, protruding into the lumen (Figure 5-1). However, endothelial cells from different vascular beds vary considerably in phenotype. Thus, in different areas of the circulatory system, the endothelium is characterized by anatomically distinct features, which allow it to adapt to the varying regulatory functions of different organs. Depending on the presence of intercellular junctions, endothelia can be classified as “continuous,” “fenestrated,” or “discontinuous.” For example, in the brain a continuous endothelial monolayer is part of the blood–brain barrier,8 whereas in the renal glomerulus, fenestrated endothelia aid in maintaing the kindey’s functions in filtration, secretion, and reabsorption of small and large molecules.9 Discontinuous endothelia can be found mainly in the liver, bone marrow, and spleen, where many cells migrate from the blood stream into the tissue and back physiologically (Figure 5-2). Moreover, endothelia from arterial and venous blood vessels also vary both in phenotype and in function. Adapting to high shear stress in the arterial vascular bed, endothelial cells from arterioles are generally elongated in the axis of the blood flow, reaching a width-to-length ratio of 1:7 in rat tracheal mucosa, whereas capillary (1:5) and particularly venous (1:2.4) endothelial cells are rounder.10 Also, endothelium-dependent vasorelaxation—one of the most important features by which the endothelium actively controls locoregionary blood flow—are generally more pronounced in arteries than in corresponding veins (see later).11 However, when venous vessels are transplanted into the arterial system (like it happens in venous coronary bypass grafts), the endothelium in these bypass vessels is flexible enough to rapidly adapt its phenotype to the novel microenvironment.
FIGURE 5-1.
(A) Electron microscopic image of a small arteriolar vessel with an endothelial cells spanning the complete inner surface of the arteriole. The endothelial cells are spread out so thinly at the vessels inner, luminal lining that the vessel wall is significantly thicker where the endothelial cell nucleus is located. Original magnification 1:25 000. (B) Another example of an endothelial cell lining the inner lumen of a blood vessel. The protrusion of the nucleus into the lumen and the many vesicles and pits that line the endothelial cell membrane are clearly seen, exemplifying this cell type as one of major metabolic activity. Original magnification 1:20 000.
The microphographs were kindly provided by Prof. Udo Schumacher, Institute of Anatomy and Experimental Morphology, University Medical Center Hamburg-Eppendorf, Germany.

The endothelium is far from being an inert, wallpaper-like inner lining of the vessel surface: quite in contrast, many regulatory functions that critically influence the vascular homeostasis are regulated in the endothelium. Some of the most potent vasoconstrictors and vasodilators interact with each other in the fine tuning of vascular tone. The hemostatic and fibrinolytic systems are controlled by endothelium-based mechanisms. Hormonal and paracrine systems such as the renin–angiotensin–aldosterone system, the eicosanoid pathways, the coagulation and fibrionolytic systems, and the nitric oxide (NO) pathway are tuned at the endothelial level. Finally (and by these biochemical means), the endothelium critically steers the activation, adhesion, and aggregation of blood platelets and the interaction of specific subtypes of leukocytes with the endothelium.
Thus, the delicate balance between the two functions as a transducing surface and as a barrier between blood and interstitium critically depends upon the structural and functional integrity of the endothelial monolayer, which must be able to adapt to changing hemodynamic situations and tolerate to a certain degree a variety of adverse conditions such as ischemia, hypoxia, and exposure to oxidants. Interaction of endothelial signalling pathways with such changes in the environment are crucial in the prevention or progression of vascular disease in a way that many vascular diseases actually start as an endothelial dysfunction. Moreover, the endothelium is able to respond to injury with specific repair mechanisms including angiogenesis and reendothelialization of a denuded vascular intima.
With a few exceptions, such as the liver, the kidney, the adrenals, the chemoreceptor trigger zone in the brain, and the bone marrow sinusoids, endothelial cells form a selective barrier between blood and tissue. This barrier function can be altered under specific conditions, such as inflammation and neovascularization. Thus, the concept of endothelial permeability is not uniform and depends on the vascular area and the (patho-)physiological context.
Why, for example, do large molecules penetrate poorly into a tumor which is otherwise characterized by leaky blood vessels?12
Why are postcapillary venules the major location of extravasation of macromolecules during inflammation,13 if it is the capillaries that are the part of the vascular tree where tubular structures aid in the exchange of macromolecules?14
And further, what is the mechanism by which even capillary microvessels become leaky in angiogenesis?
The diverse functional properties of the endothelium require active changes in cellular shape and at the same time require the generation of internal isometric forces appropriate to counteract changing hemodynamic loads acting on the monolayer.
It has been long since the discovery that the endothelial monolayer is covered by a coat of membrane-bound molecules, including glycolipids, glycoproteins, and proteoglycans, which in their totality have been named the endothelial glycocalix (Figure 5-3A). The glycocalix shows an average thickness of approximately 60 to 110 nm, which is in line with the assumed length of typical glycoproteins and proteoglycans.15 Prominent examples of molecular components of the glycocalix are components of the coagulation and fibrinolytic system like tissue factor and plasminogen, and cell adhesion molecules such as selectins and integrins, which are involved in cell–cell interactions during immune reactions and inflammatory processes. Experimental data suggest that besides this layer of large molecules that are anchored in the endothelial plasma membrane, an additional layer of macromolecules covers the endothelial surface which are not tightly fixed in the membrane of endothelial cells, but which are kept in place by molecular interactions with the glycocalix. This thick stationary layer is called the endothelial surface layer (Figure 5-3B).1 In their entirety, the glycocalix and the endothelial surface layer constitute the first line of the blood–tissue interface and are thus involved in a substantial number of physiological processes regulated by the endothelium: among these are the impact of mechanical stress on endothelial cell physiology and endothelial regulation of vascular tone, regulation of coagulation and fibrinolysis, blood cell–endothelial cell interactions during inflammation and atherogenesis, angiogenesis, and others.3
FIGURE 5-3.
The endothelial glycocalyx and surface layer. This thick stationary layer is composed of the endothelial glycocalyx and an additional layer of macromolecules that are not tightly fixed in the endothelial cell membrane. (A) The glycocalyx consists of glycoproteins and heparan sulfate proteoglycans. Glycoproteins (like integrins, selectins, and members of the immunoglobulin superfamily) are characterized by short and branched carbohydrate side chains, while proteoglykans exhibit long unbranched side chains. (B) A compex three-dimensional array of soluble plasma components including a variety of proteins, glycosaminoglycans, and hyaluronan forms a thick layer attached to the glycocalyx at the luminal side. Components of this layer dynamically exchange with the flowing plasma, but are relatively decelerated by adhesion to this layer.

The endothelium plays a crucial role in the vasodilator response to a large variety of physiological situations as well as numerous vasoactive drugs. In 1980 Furchgott and coworkers discovered that the presence of an intact endothelium is mandatory for the relaxation of isolated blood vessels by acetylcholine in vitro, whereas acetylcholine induced contractions in arterial preparations in which the endothelium had been injured or mechanically removed.6 It was soon discovered that endothelial cells release an unknown soluble relaxing factor, which was named endothelium-derived relaxing factor7 and was shown to be virtually identical with NO in 1987,16 in response to a variety of pharmacological and physiological stimuli (Figure 5-4). NO is involved in a wide variety of regulatory mechanisms of the cardiovascular system, including vascular tone. In fact, it is regarded by many as the major mediator of endothelium-dependent vasodilation. It also regulates vascular structure (inhibition of smooth muscle cell proliferation) and cell–cell interactions in blood vessels (inhibition of platelet adhesion and aggregation; inhibition of monocyte adhesion). Thus, NO plays a crucial role in the endothelium-mediated regulation of vascular homeostasis (Figure 5-5).17 Dysfunction of the endothelial NO pathway is a common mechanism by which several cardiovascular risk factors mediate certain deleterious effects on the vascular wall. Among these are hypercholesterolemia, hypertension, smoking, diabetes mellitus, homocysteine, and vascular inflammation.18,19,20
FIGURE 5-4.
Schematic representation of the l-arginine–nitric oxide pathway in the vascular wall. ADP, adenosine diphosphate; A 23187, calcium ionophore A 23187; l-NAME, Nω-nitro-l-arginine-methyl ester; l-NMMA, Nω-monomethyl-l-arginine; cGMP, cyclic 3′, 5′-guanosine monophosphate.
Reproduced with permission from Böger RH. Asymmetric dimethylarginine (ADMA): a novel risk marker in cardiovascular medicine and beyond. Ann Med. 2006;38:126-136.

FIGURE 5-5.
Nitric oxide (NO) exerts pleiotropic effects on the cardiovascular system. NO has been shown to induce endothelium-dependent vasodilation, and to inhibit platelet aggregation, leukocyte adhesion, and smooth muscle cell proliferation. NO also exerts antioxidant effects, resulting in reduced superoxide radical generation and diminished oxidation of LDL cholesterol. As all of these mechanisms are known to contribute to the pathogenesis of atherosclerosis. NO is called an “endogenous anti-atherogenic molecule.” Any condition that will reduce endothelial NO production may therefore promote atherosclerosis.
Reproduced with permission from Böger RH. The emerging role of ADMA as a novel cardiovascular risk factor. Cardiovasc Res. 2003;59:824-833.

NO activates vascular smooth muscle soluble guanylate cyclase, increasing cyclic GMP and decreasing intracellular Ca2 + concentrations. The enzyme converting l-arginine to NO was purified and cloned in 1991 and was named nitric oxide synthase (NOS).21 This enzyme releases NO from the terminal guanidino nitrogen group of l-arginine, producing l-citrulline as a by-product; it is inhibited by analogs of l-arginine that are substituted at the terminal guanidino group, like Nω -monomethyl-l-arginine (l-NMMA), Nω -nitro-l-arginine (l-NNA), or Nω -nitro-l-arginine methyl ester (l-NAME). NOS is present in at least three isoforms mainly in three types of tissues: in central and peripheral nervous tissues a constitutive isoform was isolated (NOS I), in activated macrophages an inducible NOS (NOS II) participates in unspecific host defence, and an endothelial isoform (NOS III) that constitutively releases NO is involved in the regulation of vascular tone and blood pressure. The characteristics of the three isoenzymes have been reviewed in detail by Förstermann et al.22 NO has a very short half-life, which has been determined in experimental settings to be approximately 3 to 5 seconds.23 Because of its chemical nature as a radical, it reacts with a variety of other chemical entities.24
The basal release of NO from endothelial cells produces a constant, active vasodilator tone that antagonizes a variety of vasoconstrictor mediators.25 This basal NOS activity is maintained mainly by flow-induced shear stress that is exerted by the force the viscous blood exerts on the endothelial lining while it is flowing past this monolayer.26 As any luminal narrowing of arterial vessels locally increases blood flow velocity, this may in turn induce the release of NO and contribute to poststenotic vasodilatation—a phenomenon well known in the clinical setting. Flow-induced vasodilatation also occurs during physical exercise, and NO has been shown to mediate exercise-induced vasodilatation. Acute physical exercise is also a potent stimulus for systemic NO production in humans, as indicated by the observation that the urinary excretion rates of the NO metabolite, nitrate, and its second messenger, cyclic GMP, are doubled after a 30-minute submaximal exercise in healthy subjects.27 Moreover, chronic exercise has been shown to induce endothelial NOS gene expression in dogs. The molecular mechanism behind the shear stress-induced release of NO is the phosphorylation of several serine and threonine residues in the NOS protein. Phosphorylation of Ser1177 occurs in response to endothelial cell activation as well as hemodynamic stimulation. This serine is located in the reductase domain of the enzyme. By contrast, Thr495, which is located in a site that binds an essential cofactor, calmodulin, is constitutively phsophorylated and becomes dephosphorylated upon endothelial stimulation. The dephosphorylation of this threonine residue facilitates the Ca2 +-dependent association of calmodulin with endothelial NOS, which is regarded as one important mechanism of NOS activation (for review, cf. Fleming and Busse28).
Besides fluid shear stress, a number of chemical entities can also activate endothelial NOS. Among them are acetylcholine (which, when the endothelial cells are dysfunctional, may alternatively bind to muscarinergic receptors on the vascular smooth muscle cell membrane and thereby induce vasoconstriction), thrombin, serotonin, adenosine diphosphate, and bradykinin. The common mechanism by which these chemical stimuli activate NOS is the increasing intracellular Ca2 + concentration.
NO is not the only vasodilator mediator that is actively secreted by the endothelium into the circulation and into the adjacent tissue, preferably the vascular smooth muscle cell layer; shear stress and cyclic stretch of the vessel wall are also known to stimulate the formation of the vasodilator autacoids, prostacyclin, and the endothelium-derived hyperpolarizing factor (EDHF). Prostacyclin is the major product of cyclooxygenase activity in endothelial cells. Like NO, it not only vasodilates vascular smooth muscle but also exerts an inhibitory effect on thrombocytes, thereby acting as an antithrombotic autacoid. Recent research has shown that the main isoform of cyclooxygenase that is expressed in endothelial cells even under noninflammatory conditions is COX-2—an observation that may help to explain the prothrombotic effects of the so-called selective COX-2 inhibitors and possibly also the classical nonsteroidal anti-inflammatory drugs.29
EDHF is a term that applies not just to a single factor but rather to a variety of mechanisms inducing vasodilation via hyperpolarization of the endothelial cell membrane.30 Currently four molecular mechanisms are discussed to account for EDHF-mediated responses: (a) the efflux of K+ from endothelial cells that activates either the Na+ K+ ATPase or inwardly rectifies K+ channels on smooth muscle cells; (b) generation of hydrogen peroxide (H2O2); (c) generation of a vasodilator epoxyeicosatrienoic acid by a cytochrome P450 epoxygenase; or (d) transmission of an electrical signal via gap junctions and the formation of intracellular cyclic AMP. Most of these potential mechanisms accounting for EDHF activity are difficult to study, and they may occur in parallel in the same or in different segments of the vascular tree and, so their relative contributions to the regulation of vascular tone are still not entirely clear.
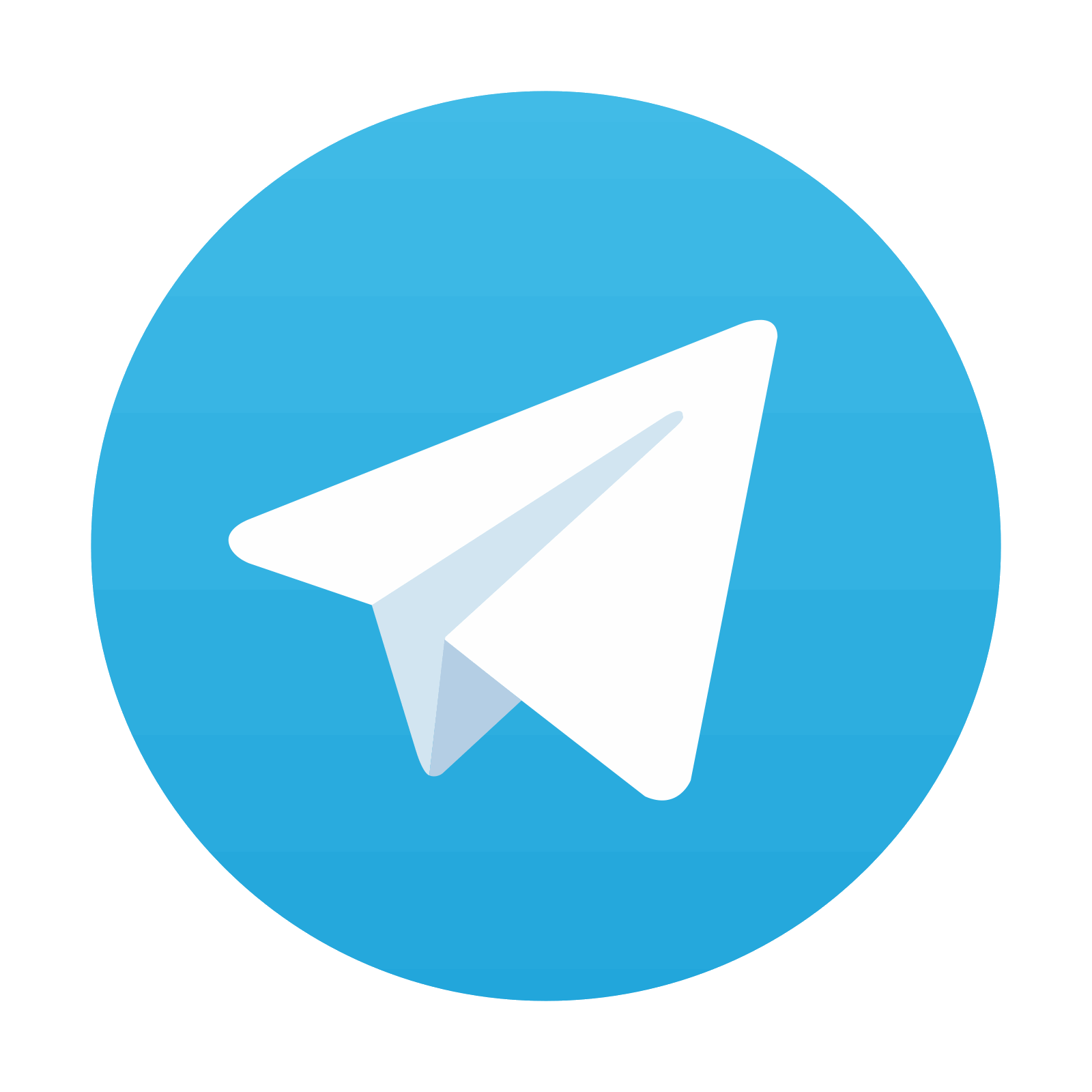
Stay updated, free articles. Join our Telegram channel
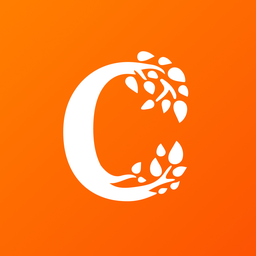
Full access? Get Clinical Tree
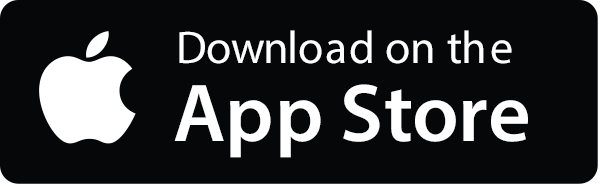
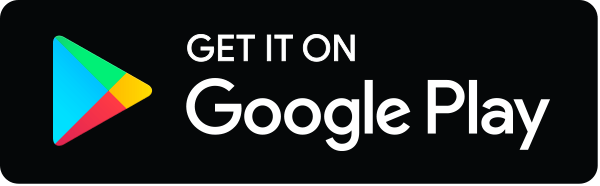