Outline
Cellular and Molecular Determinants: A View From 30,000 Feet, 136
Measuring Systolic Function: A Primer on Pressure-Volume Relations, 137
Beat-to-Beat Regulation of Systolic Function, 140
Acute Stretch—The Frank-Starling Effect, 140
Effect of Systolic Load—The Anrep Effect, 140
Effect of Heart Rate, 140
Integrative Measures of Systolic Function, 140
Impact of Pericardial Loading on Systolic Function, 143
Ventricular–Arterial Interaction, 143
Pulmonary Hypertension and Systolic Function of the Right Ventricle, 145
Treating Systolic Dysfunction, 146
Systolic Effects of Dyssynchrony and Resynchronization, 146
Summary, 149
Depressed systolic function is a core manifestation in nearly half of all patients with heart failure (HF). The underlying mechanisms are many and include defects in sarcomere function (see Chapter 2 ), abnormal excitation-contraction coupling and calcium homeostasis, ion channel dysfunction (see Chapter 1 ), mitochondrial and metabolic abnormalities, depressed cell survival signaling, enhanced autophagy and mitophagy, abnormal proteostasis, redox pathobiology (see Chapter 8 ), inflammation (see Chapter 7 ), signal transduction abnormalities, and vascular insufficiency. Systolic depression is also impacted by cross-talk between cells and signaling from the cardiac interstitium and muscle cells. Lastly, there are external factors, such as abnormal venous (preload) and/or arterial (impedance) loads, pericardial constraints, neurological controls, and other factors, which can potently influence net systolic performance.
The most direct demonstration of systolic depression in heart muscle is obtained from isolated muscle or myocytes in which sarcomere function, calcium transients, and force are measured. Human data largely stem from explanted hearts from transplant recipients or myocardium removed at time of ventricular assist device implantation. Data from membrane skinned human myocytes obtained from heart biopsies are suitable for sarcomere function studies but not the analysis of intact cell behavior. The results of such studies are discussed in detail in Chapter 2 . The inability to study intact myocytes from biopsies has proven to be a major limitation, as common forms of heart disease, including HF with a preserved ejection fraction (EF) and mutations of the dystrophin-sarcoglycan complex, are not treated with heart transplantation, and ideal animal models remain lacking. Thus, our understanding of human myocyte biology in these syndromes is sketchy at best.
Chamber systolic function is routinely assessed using strain-based measures, such as fractional shortening or EF, tissue Doppler measures, and simple pressure parameters such as the peak rate of pressure rise (dP/dt max ). These measures are not specific to underlying systolic dysfunction, nor are they capable of identifying the type of underlying defects. This is not just of academic concern as these ambiguities have undoubtedly contributed to the rather disappointing results of efforts to improve systolic function to date. EF is the most common measure used, yet it lacks both specificity and sensitivity to changes in underlying contractility. Drugs dosed to enhance EF may risk overshooting a safety margin in the effort to provide a clear quantitative change. The current classification of HF based on EF (e.g., HF with a reduced or preserved EF) is historical and convenient but also arbitrary, and unfortunately it misses critical features of underlying systolic function. Even hearts with reduced EF can have markedly disparate myocardial properties; an infarcted heart could reflect loss of a region of the wall replaced by scar, whereas another heart could reflect diffuse cardiodepression.
Here, we review our current understanding of the various mechanisms underlying systolic depression in the failing heart, the relationship between properties determined by the muscle and dysfunction as assessed in the chamber level, how systolic function is best measured in the intact heart and its relation to loading conditions, cardiac-vascular interactions and their influence on systolic function in both left and right ventricles, and lastly newer translational efforts to treat systolic dysfunction.
Cellular and Molecular Determinants: A View From 30,000 Feet
The greatest advances in understanding of HF over the past decade have come from the elucidation of its molecular-cellular determinants and their impact at the organ level. Most of this work comes from studies in mice with genetic manipulations, and is discussed in some detail in Chapter 1 .
Systolic force generation starts at the level of the actin-myosin cross-bridge, which, in turn, is coupled via structural proteins to the surface membrane to transduce into net chamber contraction. Sarcomere proteins play a central role in systolic dysfunction revealed by HF causing mutations that depress molecular motors and the myriad of posttranslational modifications that alter their function. Mouse models recapitulating sarcomere defects that induce dilated cardiomyopathy (DCM) exhibit reduced power generation and loss of function of the actin–myosin cross-bridge. Mutations in titin are common, perhaps reflecting its enormous size, and are associated with DCM. The most frequent mutations inducing hypertrophic cardiomyopathy are in myosin-binding protein C (MyBP-C), though they can also induce dilated HF. The phosphorylation of troponin I (TnI), tropomyosin, myosin light chain, MyBP-C, and titin also impacts contractility (see Chapter 2 ). Troponin I and -T phosphorylation modulates myofilament calcium sensitivity, whereas MyBP-C phosphorylation is required for β-adrenergic stimulated contractility. Both TnI and MyBP-C can also undergo proteolytic cleavage in the setting of ischemic injury, with the former resulting in a loss-of-function peptide, and the latter in a poison peptide fragment —both inducing DCM.
HF also entails disruption of intermediate filament proteins that structurally couple the sarcomere to the cell membrane. Loss of function mutations in muscle limb protein, plasma membrane sarcoglycan-dystrophin complex, focal adhesion complexes, including vinculin and metavinculin, and nuclear or mitochondrial membrane linking filaments, such as laminin, lamin, and desmin, are associated with DCM.
Another major cause of systolic impairment are abnormalities of calcium homeostasis (see Chapter 1, Chapter 2 ). This involves ion channels at the plasma membrane and intracellular proteins and calcium storage systems such as the sacroplasmic reticulum (SR). Cycling of calcium via the SR is regulated by phospholamban (PLN) through its control of the SR calcium ATPase (SERCA), but other proteins are involved, including histidine-rich calcium-binding protein, HS-associated protein X-1 (HAX-1), and heat shock protein 20. Posttranslational regulation PLN by phosphatase PP1c is controlled by inhibitor I-1, and reduced I-1 levels observed in failing hearts results in dephosphorylation of PLN and systolic depression. Abnormal activation of protein kinase C α that inhibits I-1 has been proposed as a mechanism. Hyperactive calcium release from the SR is common in failing hearts and particularly linked to excessive calcium-calmodulin activated kinase II phosphorylation of the ryanodine receptor. Other modifications of calcium-handling proteins including oxidation, nitrosylation, and SUMOylation also impair excitation-contraction coupling in the failing heart. Another set of nonvoltage gated nonselective cation channels known as transient receptor potential channels can cause abnormal mechanosensing and prohypertrophic/fibrotic signaling. Relevant species in the heart include TRPV2, TRPC1, TRPC3, and TRPC6.
Molecular signaling abnormalities are vast in the failing heart, and as an increasing number have been manipulated by genetic gain- and loss-of-function studies, their role in contractile failure has been revealed. Beyond specific genes and proteins, broad epigenetic transcriptional regulators such as BET-bromodomains (gene readers), nonprotein coding messenger RNAs (mRNAs) including microRNA (miRNA), long-non-coding RNA, and circular RNAs are also important. Lastly, the global biochemical milieu that the failing heart operates within has itself become a focus of attention. The presence of obesity, with diabetes and proinflammatory conditions, is changing the metabolic and signaling conditions in which even an otherwise “healthy” heart operates. Changes in high-energy phosphate metabolism and fuel substrate utilization have a major impact on systolic function and reserve (see Chapter 17 ).
Lastly, it is important to note that systolic dysfunction extends well beyond the myocyte, engaging the extracellular matrix, and the organ systems to which the heart is coupled. Studies suggest inadequate vasculogenesis to match demands of a hypertrophied ventricle contributes to dysfunction. MiRNAs expressed only in fibroblasts can impair heart function, while inversely, molecular signaling in the myocyte can potently impact interstitial fibrosis and inflammatory responses. Inflammatory modulation and matrix remodeling—often associated with metalloproteinase stimulation—can also impact myocyte performance (see Chapter 4 ). Signaling from peripheral organs, such as the kidney, lung, and liver, are known to impact heart function. Thus therapy to improve systolic function should be viewed broadly and include factors extrinsic to the myocyte itself.
Measuring Systolic Function: A Primer on Pressure-Volume Relations
EF is the most common index of contractile function though it lacks specificity and sensitivity. In fact, EF mostly reflects chamber dilation (end-diastolic volume) rather than contractility, since it is a ratio of stroke volume to end-diastolic volume, and the former is generally maintained until late-stage HF. Second, EF is sensitive to loading changes, notably afterload, but in dilated or hyperdynamic hearts, also to preload. From the 1960s to the 1990s, cardiovascular physiologists focused on developing more specific contractility measures based on various combinations of pressure, volume, or flow. By plotting simultaneous chamber pressure versus volume, Suga, Sagawa, and colleagues revealed how ventricular pressure-volume (PV) loops and relations provided a very powerful framework to dissect intrinsic cardiac contractile and diastolic properties from the loading systems to which the heart was coupled. This framework has since become the primary method used to identify more precise properties of the intact heart and separate them from those mediated by loading.
The fundamental concept underlying PV depictions of heart contraction is that heart muscle acts like a spring with a time-varying stiffness constant. The spring constant at the level of a chamber is called elastance, and the time-varying elastance (elastance is the inverse of compliance), which is easily derived from simultaneous pressure and volume measurements of a ventricular chamber, shows stiffness transitioning from diastole to systole and back again ( Fig 10.1A ). This time-varying elastance waveform shape is remarkably conserved among mammals (see Fig. 10.1B ), among patients with various forms of heart disease or acute modifications. It is also conserved among many gene-mutation models in mice. An exception is in heart muscle lacking MyBP-C. This sarcomere protein imposes a restraint on cross-bridge cycling kinetics, and is required for systolic elastance to be sustained once ejection starts. A newly therapy for HF in clinical trials, omecamtiv mecarbil, invokes a mechanism to prolong the time to peak elastance (discussed more below), and so also changes this underlying shape. Fig. 10.1C shows how this approach differs from a β-adrenergic receptor agonist (dobutamine).

Fig 10.2A displays typical human left ventricular (LV) PV data obtained at rest and during transient reduction of chamber preload volume. The loop furthest to the right shows the rest condition, and the labeling depicts end-diastole (point A), isovolumic contraction (point A-B), opening of the aortic valve (point B), ejection (point B-C), isovolumic relaxation (point C-D), opening of the mitral valve and initiation of diastolic filling (point D), and diastolic filling (point D-A). The loop width is stroke volume, the ratio of width to end-diastolic volume is EF, and the loop area is external (or stroke) work. When ventricular preload is rapidly reduced, both stroke volume and systolic pressure decline with each ensuing beat. This is the Frank–Starling relationship. Indeed, the same data can be used to generate typical Frank–Starling curves by plotting end-diastolic pressure for each beat versus the respective stroke volume (or cardiac output). The loops also reveal the ventricular end-systolic elastance (Ees), the slope of the end-systolic PV relationship formed by the upper-left corners of each loop. This corner point (end-systole) is determined as the time of peak elastance (Pressure/[Volume-V o ]); where V o is the chamber end-systolic volume at zero pressure. The collection of points from multiple cardiac cycles at varying loads forms the end-systolic PV relationship (ESPVR). The position and slope of this relation define systolic function. An important feature of the ESPVR is its relative insensitivity to changes in vascular loading. As shown, it is generated over a range of preload, and its linearity (often but not always the case) indicates that the slope (Ees) is preload insensitive. The ESPVR is also fairly afterload insensitive, although this is not absolute. The behavior observed in the heart is also found in single cardiac myocytes, in this case plotting sarcomere length versus tension Fig. 10.2B . As in the whole heart, myocyte end-systolic stiffness is fairly insensitive to the load applied to the cell.

PV analysis facilitates the assessment of acute changes in contractility. This is shown by clinical example in Fig. 10.2C in a patient before and after receiving the calcium channel blocker verapamil by intravenous injection. The decline in contractility is depicted by the reduced Ees. As verapamil is also a vasodilator, EF did not decline, and indeed for a long time the impact of this commonly used clinical drug on contractility was underappreciated because of this. In acute settings, changes in Ees can be unambiguously interpreted as altered contractility. However, contractility changes may manifest by shifting the entire relationship upward and leftward with or without a slope change, and this also should be interpreted as a rise in contractile performance. It is the position and not solely the slope of the relation with such acute changes that is important. Ees is also impacted by ventricular geometric changes independent of underlying muscle properties, so alterations with chronic disease are not as directly equitable with contractility change. For example, with dilated HF, PV relations shift to rightward and Ees often declines. The right shift reflects chronic chamber dilation, while the slope, can decline due to dilation per se, but also due to depressed contractility. An example of this behavior is shown in Fig. 10.2D from a mouse model. There are geometric formulas that estimate myofibrillar stress and strain from pressure-dimension data, and these can provide a more chamber-geometry independent index of muscle stiffness.
Fig. 10.2A displays another key feature of PV loops, their simultaneous depiction of total vascular afterload. The diagonal line connecting the lower right to upper left is known as the effective arterial elastance (Ea), and it incorporates both mean resistance and pulsatile vascular loading imposed on the heart during systole. Ea is calculated from the ratio of end-systolic pressure/stroke volume. It is not synonymous with vascular stiffness; its value is actually most influenced by mean arterial resistance and heart rate (Ea = ESP/SV ≈ R × HR). We do not typically think of HR as an “afterload” but, for any given arterial resistance, a pure rise in HR increases the systemic blood pressure as cardiac output increases. The net effective systolic afterload imposed on the heart is thus greater. However, unlike arterial blood pressure, Ea is minimally altered if only cardiac preload is changed (note how in Fig. 10.2A , diagonal lines from beats with different preloads remain parallel; e.g., Ea is the same). Clinical use of Ea and Ees to assess heart-vascular interaction have shown changes with aging, disease, and drugs, and as a marker of cardiovascular risk. Such analysis also has been extended to the right ventricle (RV) in patients with pulmonary hypertension.
There are some caveats to the ESPVR and its mathematical analysis. First, while the relation is often linear over a constrained range of physiological loading, its overall shape is more often nonlinear, and concaves downward. This is a particularly common observation in small mammals, such as mice, and it should be considered when linear fits are used. There are methods to parameterize the relation independent of a model-fit that can circumvent this problem. Ees is also impacted by noncontractile properties such as chamber geometry, hypertrophy, and interstitial fibrosis, inflammation, and edema. Ees rises with aging in conjunction with arterial stiffening, though this more likely reflects changes in passive than systolic-developed stiffness. Cardiac hypertrophy also manifests by a rise in ventricular Ees. This can reflect hypercontractility, but similar behavior is found in hearts with gene mutations inducing hypertrophy with depressed sarcomere function, as the hypertrophy can still stiffen muscle in the absence of abnormally enhanced contraction.
Beat-to-Beat Regulation of Systolic Function
There are three primary mechanisms that regulate beat-to-beat systolic performance of cardiac muscle. They involve the dependence of systolic force on (a) sarcomere length at the onset of contraction, (b) tension imposed during contraction, and (c) beat frequency. In the intact heart, these components translate to the impact of chamber end-diastolic volume (preload), systemic vascular impedance or wall stress (afterload), and heart rate on cardiac systolic function.
Acute Stretch—The Frank-Starling Effect
With an abrupt length increase, cardiac myocytes and muscle display an immediate rise in force without corresponding changes in intracellular calcium. This is the essence of the behavior shown by the multiple loops at varying preload in Fig. 10.2A . This response was first attributed to changes in actin-myosin filament overlap, but the marked steepness of the relation between force and sarcomere length, and its variability with contractile states, led to abandoning this hypothesis.
In 1982, Hibberd and Jewell revealed a left shift of the steady-state force-Ca 2+ relationship with increasing length, both at submaximal and maximal calcium activation, establishing length-dependent Ca 2+ activation as a central mechanism. However, the underlying mechanism for this has remained uncertain. One theory is that muscle lengthening reduced interfilament spacing between actin and myosin (i.e., stretch in one direction, compressed spacing in the orthogonal direction), favoring cross-bridge formation. The most recent work supports a critical role of titin in potentiating the recruitment of rested-to-ready strongly bound cross-bridges. Stretch extends the I-band region of titin, a protein that spans the entire sarcomere and binds both actin, myosin, and myosin binding protein C (MyBP-C). As titin is obliquely oriented to the sarcomere axis and attaches to both myosin and MyBP-C, it imposes a passive strain to alter the geometry of the thick-filament proteins. This favors the population of more force-generating cross-bridges. The magnitude of this effect rises with systolic activation, recruiting more such cross-bridges.
Effect of Systolic Load—The Anrep Effect
The Anrep or slow force response (SFR) is observed in muscle exposed to an abrupt increase in systolic load. Unlike the Frank-Starling response that is immediate and occurs without a change in myocyte intracellular Ca 2+ , the SFR evolves over several minutes and is coupled to increased Ca 2+ . It can be measured by a rise in elastance (or ESPVR left shift) or increase in muscle force. The precise source of calcium and its controlling mediators remain the subject of investigation. One culprit are members of stretch-activated channels (SACs) that augment intracellular Ca 2+ (or Na + ) and thus contraction, with TRP channel members playing a role. As previously mentioned, cardiac muscle expresses various stretch-responsive TRP channels, including TRPC1, TRPC3, TRPC6, and TRPV1, and they play a role in acute and chronic responses to afterload increase. Seo and colleagues first reported that the SFR is depressed in myocytes lacking TRPC6 (but not TRPC3). Moreover, they found the SFR is potently suppressed by activation of threonine/serine cGMP-stimulated kinase (PKG). This effect required the presence of TRPC6 that can be phosphorylated by PKG to block conductance ( Fig. 10.3A, B ). Intriguingly, the SFR is greatly increased in myocytes from a mouse model of Duchenne muscular dystrophy (e.g., lacking dystrophin and utrophin), and this is consistent with hyperactive TRPC6 mechano-responses. TRPC6 is activated by diacylglycerol coupled to G q/11 protein coupled receptors, such as the angiotensin type 1 receptor. This receptor is itself mechano-sensitive and could thereby contribute to the Anrep response.

Another explanation for the SFR attributes Ca 2+ entry to reverse mode Na + -Ca 2+ exchange (NCX) triggered by a rise in intracellular Na 2+ . This model starts with stretch activation of a Gq-receptor, the endothelin type-A receptor coupled to AT1 ligand-independent activation, that in turn stimulates PI3K and Akt. The cascade involves transactivation of epidermal growth factor receptor (EGFR) to stimulate ERK phosphorylation and activate the surface membrane Na + /H + exchanger to elevate intracellular sodium. Na + is then exchanged for Ca 2+ by the NCX, leading to the rise in force. How this mechanism would be blocked by PKG remains unclear.
The relevance of the SFR in the intact heart and to HF relates to its role in maintaining cardiac output when the heart confronts a rise in afterload stress. For example, a rise in systemic resistance is countered by an increase in cardiac contractility so that stroke volume (and in turn cardiac output) are maintained. This process, termed homeometric autoregulation in the 1950s by Stanley Sarnoff, would be particularly important in a failing heart that displays a greater decline in ejected volume at higher afterload.
Effect of Heart Rate
Systolic function of cardiac muscle is also influenced by beat frequency. This occurs within a single cycle and is the consequence of altered myocyte Ca 2+ entry (more depolarizations/minute of the L-type Ca 2+ channel and thus greater net Ca 2+ entry) and increased cycling of Ca 2+ into and out of the SR. The latter not only is intrinsic to SR calcium handling kinetics but also is controlled by rate responsiveness of Ca 2+ -calmodulin dependent kinase II. In humans, raising heart rate alone from 70 to 150 per minute increases LV contractility by 100%, and similar ranges are observed in large mammals. Mice, who operate at ∼500 per minute at rest, display a much smaller change in contractility from heart rate increase. Assessing how heart rate impacts contractility requires measures insensitive to loading (e.g., Ees), as chamber filling and effective afterload both vary with heart rate (inversely and directly, respectively). Importantly, contractility dependence on heart rate is markedly blunted in dilated and hypertrophic heart disease as a primary manifestation of depressed SR calcium handling (see Chapter 1, Chapter 2 ). Fig. 10.3C shows this phenomenon in an intact canine model of dilated HF. Increasing heart rate augments contractility (indexed by Ees) in the normal heart, but this is depressed in a dilated failing heart. Costimulation with a β-adrenergic agonist (dobutamine) further enhances HR-augmentation of contractility, since PKA-dependent phosphorylation of the L-type Ca 2+ channel and PLN (among other proteins) themselves elevate intracellular Ca 2+ . The failing heart has both depressed β-adrenergic signaling and SR function, so this combined augmentation is depressed. Failure of the force-frequency dependence is important with exertion when both sympathetic and chronotropic stimulation normally occurs.
Integrative Measures of Systolic Function
There are many other measures of systolic chamber function that are commonly used to index contractile function. These can be divided into measures of early isovolumic contraction (e.g., dP/dt max , isovolumic contraction time); early-mid ejection phase (e.g., maximal ventricular power, flow acceleration, peak strain velocities, peak velocity of shortening-stress dependence); and late systolic parameters (e.g., stroke work-derived indexes, Ees). To an extent, each of these is influenced by intrinsic systolic properties of the heart muscle, its integration into the chamber, and the loading system to which the heart is coupled. There really is no precise measure of “contractility”—a term that is itself more conceptual rather than physical. Furthermore, not all measures of chamber function index the identical behavior, and there can be discrepancies among these measurements. This is particularly true in mouse models where genetic engineering can decouple components of systolic contraction from one another.
Chamber systole begins with isovolumetric contraction, and is most often quantified by the maximal rate of pressure (or force) development. The peak first derivative of pressure (dP/dt max ) is one of the most common measures of chamber systolic function, largely due to its simplicity and ease of determination (just need pressure). It is also arguably the least directly relevant to net ejection performance by the heart, as it occurs before the aortic valve opens. dP/dt max is typically depressed in cardiomyopathy, and its absolute level found in human HF is remarkably consistent, being near 1000 mm Hg/sec (normal being near 1600–1800 mm Hg/sec). It remains a very commonly used parameter in mouse studies. A major caveat to dP/dt max is its strong preload dependence. In mice, a change of as little as 2 to 3 microliters of end-diastolic volume (10% decline) can markedly alter dP/dt max ( Fig. 10.4A ). This dependence is minimized by regressing dP/ systolic dt max from multiple beats at varying end-diastolic volumes versus EDV from the same cycles. While this approach is rarely used clinically due to its complexity, it is easily employed experimentally. dP/dt max is also influenced by internal loading (such as reflected by intermediate filaments, microtubules, and titin), the extracellular matrix (i.e., edema, inflammation, collagen dysregulation), the phosphorylation state of MyBP-C, sarcomere cross-bridge properties (e.g., cooperativity upon activation), and the dynamic coordination of contraction by different portions of the cardiac chamber wall. The latter is rendered abnormal in the presence of conduction delays, such as a left bundle branch block (LBBB), and the resulting discoordination depresses dP/dt max even without any primary change in contractile function of the myocytes. When one side of the heart contracts early while the opposite side remains in diastole, pressure force is dissipated by stretching the still-relaxed wall, and the rate of pressure rise is slowed. Acute increases in dP/dt max associated with biventricular stimulation of a dyssynchronous failing heart occur within a single beat, and similarly do not indicate a change in underlying contractile function, but rather chamber-level consequences of improved coordination of wall contraction.

Early-to-mid systolic parameters reflect times when shortening velocity (e.g., wall motion correlated to peak flow from the heart) and flow is maximal. Peak velocity of chamber shortening varies inversely with wall stress, and this hyperbolic relation has been used as a measure of contractility (see Fig. 10.4B ). Noninvasive image-based measures include tissue Doppler peak systolic velocity (see Chapter 32 ), or load-incorporating variants, such as maximal ventricular power (needs to be adjusted for preload volume), or the circumferential shortening velocity adjusted for wall stress. Maximal power is the peak product of ventricular pressure and outflow, and can be derived noninvasively from Doppler flow imaging and cuff/tonometry-derived blood pressure data. Maximal power is then adjusted for chamber end-diastolic volume to derive a parameter with little load dependence. The latter is not the case if afterload changes are marked, as may apply to the right heart in severe pulmonary hypertension. This index displays far more marked sensitivity to contractile function than does the EF, and can be measured during exercise stress procedures.
Tissue Doppler imaging has given rise to strain and strain-rate analysis. These approaches essentially quantify myocardial wall motion—as obtained from magnetic resonance imaging. Actual regional stresses remain unknown, yet these also influence measured strains and strain rates. Nonetheless, strain rate has been found to correlate with dP/dt max and indices derived from end-systolic PV relations (see Fig. 10.4C ), and clearly is prominently influenced by chamber systolic function. In genetic models of hypertrophic cardiomyopathy, tissue Doppler has been used to define early abnormalities of chamber function that precede the evolution of cardiac hypertrophy. Tissue Doppler has been widely employed to index contractile discoordination in patients with cardiac failure and conduction delay.
The most commonly used late-systolic parameter is EF—the chamber translation of fractional shortening. EF is easy to measure, its value is independent of calibration errors of absolute volume assessment (i.e., it is dimensionless), it is only moderately sensitive to inotropic changes, and it is not very sensitive to pure alterations in cardiac filling volume (preload), and inversely dependent on afterload and heart rate. In human heart disease, EF mostly reflects the presence of chamber dilation/remodeling since the numerator (stroke volume) is relative preserved in many forms of heart disease until very late stage decline, whereas the denominator (EDV) can increase markedly. It is unfortunate in this regard that we continue to categorize HF by EF, including the nomenclature of HF with a reduced or preserved, and mid-range EF, insofar as these categories do not imply meaningful differences in underlying myocardial pathophysiology, molecular mechanisms, or necessarily what type of intervention is likely to be most successful.
While the Frank-Starling curve remains an important element of systolic analysis, its utility is limited by strong afterload and heart rate dependencies, and ambiguities associated with the use of end-diastolic filling pressure to index preload. An alternative approach, developed over 50 years ago, was to assess relations between cardiac stroke work and preload (preload-recruitable stroke work), with the latter indexed by end-diastolic volume rather than pressure. Stroke work (area of a PV loop) is less afterload dependent than stroke volume, as it incorporates pressure as well, and the stroke work–end-diastolic volume (SW-EDV) relation is both linear and minimally influenced by chamber load, while still reflecting systolic function. It has a distinct advantage among all the systolic function indexes in that its units are of force (the volume terms drop out from numerator and denominator). This makes it more chamber-size independent, and values of the slope of the relation lie between 75 and 90 mm Hg in normal hearts from mouse and rat up through to dog, pig, and human. As with Ees, methods to assess preload recruitable stroke work (PRSW) from single-beat data using noninvasive analysis have been reported.
Impact of Pericardial Loading on Systolic Function
The pericardium surrounds all chambers of the heart and has the capacity to alter transmural wall stress and the relation between diastolic distending pressures and volumes. This results in an important impact on the apparent Frank-Starling relation. For example, pericardial constraining forces are largely responsible for the apparent descending limb of the Frank-Starling relation in dilated failing hearts. This fall in force at high preload was classically attributed to the overstretch of the sarcomere with a loss of thick and thin filament overlap. However, this is not possible in cardiac muscle due to the complex collagen weave that surrounds each cell in the myocardial syncytium, as well as cytoskeletal membrane proteins within the myocyte. Rather, Tyberg and colleagues revealed that high preload can result in a decline in transmural pressure (not absolute chamber pressure) due to constraining forces provided by the pericardium. In patients with DCM, increased end-diastolic pressures may not be associated with elevated transmural pressure due to higher pericardial pressure. This is not the result of fluid pressure in the pericardial space, but from the membrane itself, which does not over stretch as the heart dilates. When preload is reduced, the pericardial pressure declines, so that transmural distending pressures can actually rise, filling the heart with more blood even as the apparent intracavity diastolic pressure is declining. Plots of cardiac output (CO) versus end-diastollic pressure (EDP) appear biphasic, rising at lower EDP and falling at higher EDP, whereas the same data plot as CO versus EDV is upward linear.
Ventricular–Arterial Interaction
The interaction of the heart and arterial system into which it ejects critically determines cardiac output, EF, stroke work, mechanical efficiency, systolic pressure, and many other key integrated properties. Depending upon the matching of heart to vessel, these variables can be either optimized or not. This interaction also explains differences in cardiac output and systolic blood pressure responses to changes in arterial or venous dilation or inotropy between normal, hypertrophied, and failing hearts.
Among the more successful methods to assess ventricular-vascular coupling is the one derived from the PV framework, employing Ees to index the systolic heart property, and Ea to index the arterial load. As noted in Fig. 10.2A , E a is the ratio of end-systolic pressure to stroke volume and its value is dominated by mean arterial resistance and heart rate. It is also influenced by phasic (reactive) loading properties of the arterial system; that is, properties due to wall compliance, wave reflections, inertance, etc. Coupling is then expressed by a ratio of E a to the ESPVR slope (E a /E es or E es /E a ) ( Fig. 10.5A ).

When the E a /E es ratio is near 1.0, the transfer of energy or stroke work from heart to arterial system is near optimal, and the heart operates at near optimal cardiac efficiency. In normal individuals, this ratio is near 0.8, and this is maintained during exercise, with concomitant rises in both Ees and Ea. This behavior is conserved across mammals, and may relate to an evolutionary process designed to maintain the lowest cardiac/body size ratio.
In hearts with depressed contractility, work and power output are far from optimal, as they fall with higher vascular loading (greater Ea) and myocardial depression (lower Ees). Asanoi and colleagues first reported coupling ratios in patients with normal, moderate, and severely depressed LV function, finding ratios >3 (see Fig. 10.5B ) in DCM.
The interaction of Ees and Ea provides a useful means of understanding and/or predicting the response of a given heart to a particular therapeutic intervention. A little algebra helps clarify how this works. From the definition of E es = P es /(V es −V o ) = P es (V ed −SV−V o ), where Pes, Ves are end-systolic pressure and volume, Ved is end-diastolic volume, SV is stroke volume, and Vo is the zero-pressure intercept of the ESPVR, and for Ea=Pes/SV, one can write:
Ees×(Ved−SV−Vo)=Ea×SV
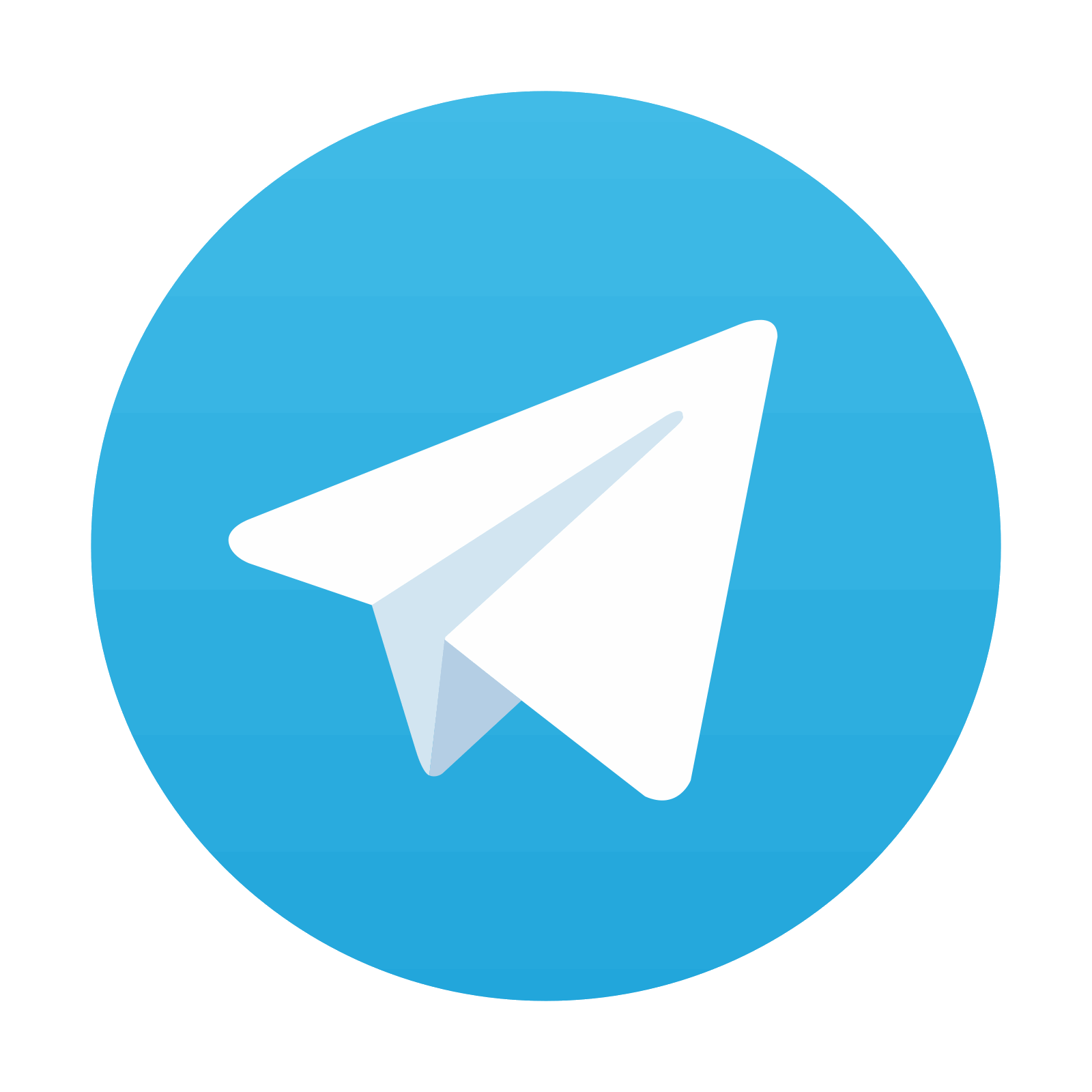
Stay updated, free articles. Join our Telegram channel
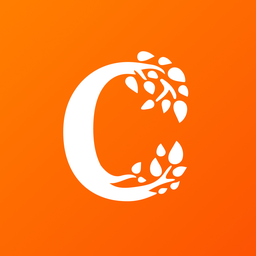
Full access? Get Clinical Tree
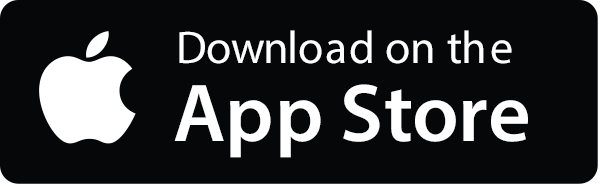
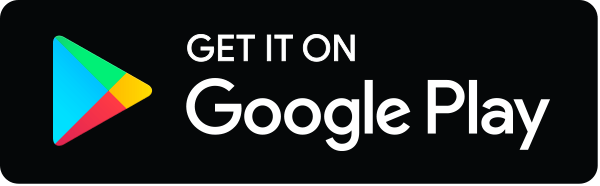
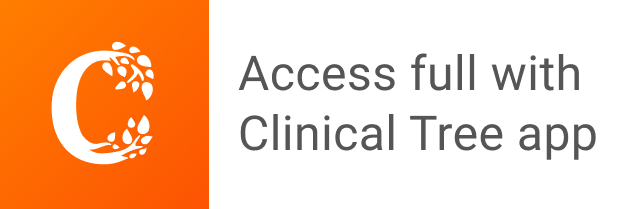