Outline
Overview of Cardiac Metabolism, 233
Hallmarks and Regulation of Cardiac Energy Metabolism, 233
Crosstalk Between Cardiac Metabolism and Signaling, 235
Significance of Cardiac Energetics for Heart Disease, 235
Metabolic Dysfunction in the Failing Heart, 235
Energy Depletion in the Failing Heart, 235
Substrate Utilization and Mitochondrial Metabolism in the Failing Heart, 236
Novel Concepts Linking Metabolic Changes and Epigenetic Changes and Posttranslational Modifications Downstream of Metabolic Intermediates, 236
Crosstalk Between Cardiac Metabolism and Systemic Metabolism, 238
Metabolic Remodeling and Risk for Heart Failure Development, 238
Methods to Evaluate Defects in Cardiac Energetics, 240
Therapeutic Approaches to Modulate Cardiac Energetics, 240
Modulation of Substrate Oxidation Patterns, 240
Modulation of ROS Detoxification and Oxidative Phosphorylation, 241
Exercise and Cardiac Metabolism, 242
Summary and Future Directions, 242
Overview of Cardiac Metabolism
Hallmarks and Regulation of Cardiac Energy Metabolism
Cardiac energy metabolism is essential to maintain cardiac pump function. To enable the heart to beat 100,000 times a day for a lifetime, the heart exhibits a highly regulated and efficient system for adenosine triphosphate (ATP) regeneration, generating up to 6 kg of ATP every day, which is 15- to 20-fold its own weight. Thus the heart is one of the most metabolically active organs, and its prolific capacity for generating energy is underscored by the very high density of mitochondria and their unique patterns of distribution relative to areas of high energy utilization such as sarcomeres (the contractile unit) and the sarcolemma where significant changes in ionic flux occurs. Under physiologic conditions, the heart generates more than 95% of its ATP by oxidative metabolism of energy substrates; 60% to 70% of ATP arises from the oxidation of fatty acids (FAs), and 30% to 40% from the oxidation of glucose and other substrates such as lactate, amino acids, and ketone bodies, depending on their availability in the circulation. Utilization of FAs for ATP regeneration initially requires FA uptake into the cardiomyocyte via fatty acid transporters such as CD36 and the fatty acid transporters (FATP, family of proteins). Following esterification to acyl CoA by acyl-CoA synthetase, they are imported into mitochondria by transient coupling to carnitine via the carnitine palmitoyltransferase (CPT) system. Once imported into mitochondria, acyl Coenzyme A (CoA) is oxidized in the β-oxidation spiral to yield acetyl CoA and flavin adenine dinucleotide (FADH). Utilization of glucose requires sarcolemmal glucose uptake via the classical insulin-responsive glucose transporter 4 (GLUT4) and the constitutive glucose transporter (GLUT1). Recent evidence suggests that some glucose might enter the heart via the sodium-glucose transporter (SGLT1). However, GLUT4, which shuttles to the cell surface with cardiomyocyte contraction, likely accounts for the bulk of myocardial glucose uptake. Upon entering the heart, most of the glucose is metabolized via glycolysis to yield ATP, reduced nicotinamide adenine dinucleotide (NADH), and pyruvate. Additional metabolic branches of glycolysis such as the hexosamine biosynthetic pathway (HBP) or the pentose phosphate pathway (PPP) yield metabolites that play an important role in signal transduction, oxidation-reduction REDOX regulation, and nucleic acid synthesis. Pyruvate is imported into mitochondria via the mitochondrial pyruvate transporter and decarboxylated by pyruvate dehydrogenase (PDH) to acetyl CoA. Acetyl CoA derived from metabolic precursors such as glucose, FAs, and others (e.g., ketones) enters the (tricarboxylic acid) TCA cycle. Oxidation of acetyl CoA in the TCA cycle generates NADH and FADH, which donate electrons to the electron transport chain (ETC), which pumps protons into the mitochondrial intermembrane space to generate the proton-motive force that is dissipated via ATP synthase to ultimately regenerate ATP from adenosine diphosphate (ADP) by the process of oxidative phosphorylation (OXPHOS) ( Fig. 17.1 ).

Several modes of regulation adapt cardiac energetics to acute or chronic changes in energy demand. Long-term regulation of cardiac energy metabolism is usually governed by changes in gene expression. For example, transcriptional regulators that regulate genes that encode mitochondrial enzymes of mitochondrial OXPHOS can be induced or repressed by various stimuli that correlate with changes in cardiac substrate metabolism. These transcriptional regulators include nuclear receptors that regulate genes that encode fatty acid oxidation (FAO) enzymes such as peroxisome proliferator activated (PPAR)α, PPARβ/δ, and estrogen related receptor (ERR)α, transcription factors that increase OXPHOS gene expression (transcription factor A of mitochondria [TFAM], nuclear respiratory factors [NRFs], ERRα), and transcriptional coactivators that regulate both the expression of FAO and OXPHOS genes (PPAR gamma coactivator [PGC]-1α, PGC-1β]) ( Table 17.1 ). Thus conditions of FA excess such as obesity and diabetes are associated with increased expression of transcriptional regulators of FAO, whereas heart failure (HF), which is associated with decreased mitochondrial energetics capacity is associated with repression of PGC-1α. Mechanisms that acutely regulate short-term changes in energetics include signaling pathways that drive regulatory pathways via posttranslational mechanisms. One of the best-studied pathways is adenosine monophosphate-activated protein kinase (AMPK) activation, which drives catabolic pathways and inhibits anabolic pathways by phosphorylation of specific metabolic enzymes that regulate glycolysis, glycogen synthesis, and FAO. A second pathway is protein deacetylation of a broad variety of metabolic enzymes, including FAO enzymes, TCA cycle enzymes, PDH, OXPHOS subunits, and the F O F 1 -ATPase by a family of NAD + -dependent deacylases called sirtuins, which links cardiac metabolic capacity with nutrient sensing via NAD + . Allosteric regulation of metabolic enzymes represents another important mechanism for the short-term regulation of cardiac metabolism. This is classically exemplified by the “Randle cycle,” whereby increased FA utilization inhibits glucose utilization via increased generation of citrate, which inhibits glycolysis, and the reverse Randle cycle, whereby increased malonyl CoA that may occur when glucose utilization is increased in turn allosterically inhibits CPT1 and mitochondrial FA utilization. Cardiac energy metabolism is also acutely regulated by various stress hormones (e.g., catecholamines), cytokines, insulin signaling, changes in workload, or concentrations of metabolic substrates.
Molecule | Predominant Functions in Heart | Mechanism of Action |
---|---|---|
PPARα | Increase FAO gene expression | Transcription factor |
PGC-1α, PGC-1β | Increase FAO and OXPHOS gene expression, increase mitochondrial biogenesis, ROS detoxification | Transcriptional coactivator |
ERRα | Increase FAO and OXPHOS gene expression | Transcription factor |
TFAm | mtDNA replication, OXPHOS gene expression | Transcription factor |
NRF1 | OXPHOS gene expression | Transcription factor |
AMPK | Increase FA uptake and oxidation, increase glucose uptake, increase glycolysis, inhibit anabolic pathways | Protein kinase |
SIRT1, SIRT3, SIRT5 | Increase FA and glucose oxidation, increase mitochondrial function, ROS detoxification | Deacylase |
Crosstalk Between Cardiac Metabolism and Signaling
Although the main function of myocardial energy substrate metabolism is to generate ATP, metabolic intermediates also serve as signaling molecules. Both glycolysis and mitochondrial energy metabolism generate and consume NADH and thereby participate in the regulation of the NAD + /NADH ratio, which can be considered an indicator of the cellular energy charge. Accordingly, this ratio increases during energy demand and decreases under conditions of sufficient ATP supply, which in turn regulates the activity of sirtuins, which not only regulate energetics but also cellular senescence, growth, mitochondrial biogenesis, and reactive oxygen species (ROS), among other mechanisms ( see also Chapter 8 ). In addition to entering glycolysis, glucose may also enter other pathways such as the PPP and the HBP. Flux through the HBP generates glucosamine that increases O-GlcNAcylation of many proteins that regulate diverse cellular functions. These include proteins, the modification of which modulates transcription factor activity, epigenetic regulation, cellular Ca 2+ homeostasis, cell growth, cell survival, oxidative stress, and mitochondrial function. Flux through the PPP regulates the generation of NADPH, which, as a substrate for NADPH oxidases, serves an important generator of cytosolic ROS. PPP intermediates also maintain the levels of reduced glutathione. Thus PPP flux participates importantly in the regulation of cellular REDOX. The redox state of cells plays important roles in multiple cellular signaling pathways, including oxidative modification of regulatory proteins that includes targets such as phosphatases, protein kinases (A, D, and G), cytokines, mitogen-activated protein kinases, or insulin signaling. In this regard, the activity (i.e., forward or reverse mode) of the mitochondrial nicotinamide nucleotide transhydrogenase (NNT), which transfers electrons between NAD(H) and NADP(H) to balance ATP production and antioxidant capacity, is dependent on metabolic demand and cardiac workload. Another metabolite affecting intracellular signaling is citrate, the conversion of which to acetyl CoA by ATP-citrate lyase may contribute to direct enzyme acetylation by cytosolic acetyltransferases, as well as to nuclear histone acetylation and subsequent epigenetic regulation of gene expression. The examples discussed here are not exhaustive because many additional metabolites, such as succinate, are being identified and may regulate signaling pathways within the heart under basal conditions or in response to stressors such as ischemia/reperfusion.
Significance of Cardiac Energetics for Heart Disease
Impaired cardiac metabolism and energy depletion are well recognized to contribute to cardiac dysfunction and reduced efficiency in terms of energy transfer to contractile work ( see also Chapter 2 ). These changes, which contribute to many cardiac pathologies by impairing ATP-dependent intracellular processes such as myofilament contraction and maintenance of ion homeostasis, have been described in prevalent disorders such as myocardial ischemia reperfusion injury, diabetes-related cardiac dysfunction, cardiac hypertrophy, and HF. In ischemia reperfusion, ATP regeneration is impaired due to limited oxygen and substrate supply and to persistent impairment in OXPHOS and mitochondrial integrity. In cardiac hypertrophy, substrate preference may shift toward a relative increase in glucose utilization, accompanied by early defects in mitochondrial function, whereas in HF, overall mitochondrial oxidative metabolism can be impaired. In diabetes, increased FAO and impaired glucose utilization are associated with impaired mitochondrial ATP generation, mitochondrial uncoupling, and impaired cardiac efficiency that may be characterized by increased myocardial oxygen consumption.
Recent work has revealed important sex differences in cardiac metabolism in healthy subjects and in individuals with cardiac dysfunction or high-risk conditions such as diabetes. Myocardial oxygen consumption (MVO 2 ) and myocardial FA utilization (MFAU) are higher in healthy females relative to males and may be related to estrogen. These differences persisted in individuals with type 2 diabetes (T2D) and in those with HF with preserved ejection fraction (HFpEF) ( see also Chapters 11 and 39 ). Myocardial blood flow (MBF) rates were higher in women with HFpEF, and MBF was correlated with better event-free survival. Despite lower rates of MFAU, diabetic men exhibited greater impairment in diastolic relaxation. In a study of normal, obese, and T2D subjects, diabetes and obesity reduced glucose utilization, but sex also had a powerful effect on glucose utilization, with levels of glucose utilization being lower in females. Because glucose uptake and metabolism rates were relatively low in nonobese women, they were not markedly different from those in obese and T2DM women. Thus the potentially detrimental effects of obesity and diabetes on myocardial glucose metabolism appear to be more pronounced in men than women.
The relevance of altered myocardial metabolism for disease development and progression is underpinned by a number of clinical trials that investigated the effects of specific metabolic interventions, in particular in HF. Some of these trials have yielded promising results despite small patient numbers, including improvements in ejection fraction (EF), HF symptoms, and HF hospitalization, although data from large randomized controlled clinical trials investigating hard clinical end points are lacking to date. Finally, a number of metabolic cardiomyopathies have been described in which a single mutation or enzyme deficiency may lead to cardiac failure, likely due to impairment in cardiac energetics, further emphasizing the direct relationship between myocardial energy metabolism and HF development. These defects include systemic carnitine deficiency, malonyl carboxylase deficiency, deficiency of FAO enzymes, and inherited mutations in mitochondrial (mtDNA). This chapter will review mechanisms, diagnostic approaches, and potential therapeutic strategies related to metabolic dysfunction in the failing heart.
Metabolic Dysfunction in the Failing Heart
Energy Depletion in the Failing Heart
Continuous cardiac pump function requires the regeneration of large amounts of ATP. Energy depletion is a well-established characteristic of HF irrespective of its etiology. When directly examined, energy deprivation in failing human hearts is characterized by a reduction in the phosphocreatine (PCr)/ATP ratio. The PCr shuttle transfers ATP from mitochondria to myofilaments and transfers ADP back to the mitochondria for rephosphorylation. PCr receives its phosphate group from ATP by the creatine kinase reaction, which favors ATP synthesis over PCr synthesis by approximately 100-fold. Thus, when ATP demand outweighs ATP availability, the PCr/ATP ratio declines first and represents a sensitive and powerful index of the energetic state of the heart. In HF patients, PCr/ATP was found to be reduced, and the magnitude of this reduction correlates with New York Heart Association (NYHA) functional class, systolic and diastolic function, and mortality. In addition, mitochondrial respiratory capacity and rates of ATP synthesis are markedly decreased in HF patients, both in ischemic and nonischemic dilated cardiomyopathy. An unresolved question remains as to whether impaired cardiac energetics are cause or consequence of HF. There is a large body of evidence particularly from animal studies that support a causal role, between energy depletion and the pathophysiology of HF. However, cardiac mitochondria isolated from failing hearts at the time of left ventricular assist device (LVAD) implantation might not reveal intrinsic defects, and metabolomics analysis reveals improved mitochondrial metabolism after ventricular unloading, suggesting that some degree of mitochondrial plasticity might exist in failing hearts. It is also noteworthy that animal studies that directly seek to increase mitochondrial biogenesis in HF models might not necessarily improve ventricular function in the face of ongoing hemodynamic stress. Thus it is likely that the mitochondrial changes associated with HF might reflect both an adaptive response and a direct contributor to ventricular dysfunction.
Substrate Utilization and Mitochondrial Metabolism in the Failing Heart
The metabolic alterations related to impaired cardiac energetics in HF are manifold. Although studies of substrate utilization in HF have yielded conflicting results, most studies show a decrease in FAO, which is most pronounced in the advanced stages of the disease. In parallel, a relative increase in glucose utilization (glycolysis and glucose oxidation) is frequently observed during the evolution of pathologic cardiac hypertrophy and early in HF. However, glucose oxidation is invariably markedly reduced in the later stages of the disease ( Fig. 17.2 ). Although the report of conflicting results may be related to differences in the etiology and stages of HF, the adaptive or maladaptive character of preferential glucose oxidation remains a subject of debate. Support for a beneficial role of increased glucose utilization comes from interventional studies suggesting that inducing a relative increase in glucose utilization could be beneficial, as will be discussed further later. HF-associated changes in substrate preference may be related to decreased PPARα signaling, resulting in reduced expression of FAO genes, and decreased signaling of PGC-1 coactivators that may downregulate both expression of FAO and OXPHOS genes. The mechanisms for increased glucose utilization remain incompletely understood. Increased glucose uptake and glycolysis may be related to increased GLUT1 expression and activation of AMPK. In addition, chronic or intermittent tissue hypoxia may increase hypoxia-inducible factor 1-α (HIF1α) levels, which may also increase glycolysis. Reduced FA capacity might also increase glucose utilization via the Randle phenomenon. Reduced glucose oxidation when it develops has been primarily attributed to reduced PDH activity or mitochondrial pyruvate uptake. The imbalance between the coupling of (increased) glycolysis to (reduced) glucose oxidation contributes to lactate accumulation and intracellular acidosis. Activation of the sodium hydrogen exchange and other mechanisms in turn lead to Na + and Ca 2+ overload, which places an additional energetic burden on the cardiomyocyte. The ATP requirements for restoring ion homeostasis, in addition to the ongoing need for myofilament contraction, further exacerbate impaired cardiac contractility and efficiency.

Although most studies have focused on glucose and FA metabolism, recent studies in humans and animal models have revealed that the failing heart increases ketone body utilization. Increased ketone body utilization by the failing heart could reflect an adaptation that maintains cardiac contractile function in the face of significant restriction in substrate availability from other sources. Whether or not increasing ketone body utilization by pharmacologic, dietary, or other means in the context of HF will improve cardiac function is not known but is an area of active investigation. HF is also associated with the increased de novo synthesis and accumulation of the lipid derivative ceramide, which has been proposed to be mechanistically linked to ventricular dysfunction. In vitro studies have linked ceramide accumulation to cell death and mitochondrial dysfunction in cultured cardiomyocytes. Finally, recent studies have suggested that HF is also associated with impaired catabolism of branched-chained amino acids (BCAAs). In animal models with genetic impairment in BCAA metabolism, HF ensues. The clinical utility or modulating BCAA metabolism in humans with HF remains to be determined. However, clinical trials have now been initiated in which hypoalbuminemic patients with HF have been treated with BCAA supplementation.
In the advanced stages of HF, the general impairment in oxidative capacity is due in part to defective ETC and F O F 1 -ATPase activity (see Fig. 17.2 ). Reduced expression of nuclear- and mitochondria-encoded subunits of the ETC and F O F 1 -ATPase, oxidative damage of mitochondrial proteins, lipids, and DNA, impaired activity and formation of OXPHOS complexes and respiratory supercomplexes, reduced TCA cycle enzyme activities, posttranslational protein modification (e.g., hyperacetylation, phosphorylation), increased proteolysis, decreased NNT activity, defects in mitochondrial dynamics, increased mitophagy and apoptosis, and cardiolipin remodeling are some, but not all of the mechanisms, that have been associated with impaired mitochondrial respiration and ATP synthesis in the failing heart. Decreased activity of sirtuin 1, 3, 5, 6, and 7 may contribute to HF due to overall slowing of oxidative metabolism by decreasing the removal of specific protein acetylations from a broad variety of energy metabolic enzymes (PDH, FAO enzymes, TCA cycle enzymes, OXPHOS subunits, F O F 1 -ATPase) leading to changes in their activity. Finally, increased mitochondrial oxidative stress resulting from inhibition of ROS detoxification represents another important mechanism that contributes to impaired mitochondrial bioenergetics in the failing heart.
Novel Concepts Linking Metabolic Changes and Epigenetic Changes and Posttranslational Modifications Downstream of Metabolic Intermediates
As discussed earlier, an important consequence of altered metabolic function in the failing heart is energy or ATP depletion. However, it is now becoming widely accepted that the intermediates of metabolic pathways act as signaling molecules to alter protein function and gene expression. Two areas of research where disruption of cardiac metabolism has become linked to mechanisms of HF include studies of posttranslational modifications (PTMs) and more specifically the recently expanding area of epigenetics. In fact, these areas have received so much attention that entire issues of journals have been dedicated to the role of PTMs in heart metabolism. It is also important to note that these two areas are not completely separate, because many of the PTMs that occur on contractile and metabolic proteins also contribute to epigenetics (e.g., acetylation, methylation, O-GlcNAcylation). Briefly, epigenetics includes regulation of gene expression by PTM of histones, modification of DNA (i.e., methylation and hydroxymethylation), and posttranscriptional regulation by noncoding RNAs (i.e., microRNAs [miRNAs] and long noncoding RNA [lncRNA]). Although many of the initial studies concerning epigenetics were focused on inherited changes impacting gene expression, recent work has shown that acquired alterations in epigenetic marks contribute both to disease susceptibility and progression. These observations place metabolism at the nexus of causality and consequence of epigenetic alterations; and unraveling these interactions has resulted in a number of novel insights.
Many risk factors associated with HF (e.g., obesity and diabetes) lead to excessive or dysregulated nutrient utilization that alters the concentrations of substrates or allosteric regulators that control mediators of PTMs such as histone or lysine acetyl transferases (KATs), histone or lysine deacetylases (KDACs), methyltransferases, and O-GlcNac transferase (OGT). In addition, impaired mitochondrial function that accompanies HF also independently regulates metabolites (e.g., NAD + , acetyl-CoA) that may further impact the regulation of these enzymes. For example, in human end-stage HF there is an elevation in acetyl CoA levels that correlates with increased acetylation of mitochondrial proteins. Because these modifications alter mitochondrial oxidative function, they exacerbate and perpetuate the link between energy starvation and PTMs that may both contribute to the progression of HF. Very recent evidence found that in HF there is an elevation of the miRNA, miR-195, which in turn decreases levels of the mitochondrial deacetylase sirtuin 3 (SIRT3), leading to enhanced mitochondrial protein acetylation, that ultimately contributes to mitochondrial dysfunction by dysregulating ATP synthase and PDH complex. Importantly, loss of SIRT3 activity by genetic ablation in mice was sufficient to induce mitochondrial and contractile dysfunction. In theory, this would further alter the acetyl CoA pool, leading to additional modulation of protein acetylation. These studies, along with numerous others on SIRT1 and SIRT6 and the extensive literature on protein acetylation, have made this an enticing area of potential therapeutic intervention, including regulation by a number of naturally occurring phytochemicals (e.g., resveratrol, curcumin).
Although less well studied, the serine and threonine O-linked addition of β-N-acetyl-glucosamine (O-GlcNAcylation) has emerged as an important albeit controversial factor in HF. Some of the early limitations in studies of protein O-GlcNAcylation resulted from technical hurdles in measuring site-specific modifications, many of which have recently been overcome. Unlike many PTMs, the addition or removal of which are generally mediated by numerous enzymes, O-GlcNAcylation addition and removal is regulated by single gene products, namely OGT and O-GlcNAcase (OGA), respectively. This has led to the speculation that the primary form of regulation of this pathway is the rate of flux through multiple metabolic pathways such as glucose, amino acids, FA, and nucleotide metabolism leading to accumulation of N-acetyl glucosamine or its precursors. More recently, subcellular redistribution of OGT and OGA has also been postulated to govern the regulation of protein O-GlcNAcylation.
Increased protein O-GlcNAcylation has been described in samples obtained from failing human or rodent hearts and correlates with decreased cardiac mitochondrial oxidative function, particularly in the context of diabetes. In contrast, in the context of ischemia, increased O-GlcNAcylation is associated with protection against cardiac injury, by inhibiting calcium overload and reactive oxygen species generation or by increasing cardiac stem cell survival. Interestingly, O-GlcNAcylation might not mediate cardiac hypertrophy induction in mouse models, because inducible cardiomyocyte ablation of OGT in the context of transverse aortic constriction exacerbated LV remodeling despite reduced protein O-GlcNAcylation. Similar to other PTMs, miRNAs also regulate O-GlcNAcylation pathways. miR-539, which negatively regulates OGA expression, is increased in HF, leading to increased protein O-GlcNAcylation, representing an additional potential avenue for future therapeutic regulation. More work is needed to understand the nuanced physiology of O-GlcNAcylation in the adaptive responses of the heart to stress and how disruption of cardiac metabolism in HF may perturb O-GlcNAcylation and contribute to cardiac pathology.
Additional metabolites that might not be directly involved in classical substrate oxidation are also emerging as important mediators of epigenetic modifications that could contribute to ventricular remodeling. Specifically, in HF associated with some forms of leukemia, there is a resultant increase in levels of the oncometabolite d -2-hydroxyglutarate (D2-HG), which leads to both altered flux through the citric acid cycle as well as altered histone methylation and acetylation in the heart. Furthermore, the authors showed that D2-HG is sufficient to impair cardiac performance and reduce ATP levels. Whether similar mechanisms exist in other forms of HF remains to be completely explored. As discussed elsewhere in this chapter, human HF is associated with increased ketone body utilization, which may alter tissue levels of d -β-hydroxybutyrate (BHB). These observations raise interesting mechanistic links between substrate metabolism and adverse cardiac remodeling. Cardiomyocyte-specific overexpression of the rate-limiting enzyme in BHB oxidation, d -β-hydroxybutyrate dehydrogenase I (BDH1), attenuates cardiac remodeling and dysfunction in pressure overload–induced HF, whereas cardiomyocyte-specific deletion of a related enzyme, succinyl-CoA:3-oxoacid CoA transferase (SCOT), exacerbates contractile dysfunction in the context of pressure overload–induced HF. Although some of this is likely explained by changes in metabolic substrate flux to ATP generations, a number of studies have also identified that BHB is a critical signaling molecule that also impacts epigenetic pathways.
This section has not provided an exhaustive review of the role of PTMs in HF. Additional PTMs likely play important roles in normal physiology, and their disruption in HF progression is potentially linked causally to altered cardiac metabolism in HF and in turn to the pathophysiology of LV remodeling. For example, methylation, palmitoylation, small ubiquitin-like modifier mediated modifications (SUMOylation), succinylation, and others have all been linked to regulating cellular pathways that are directly linked to cardiac dysfunction. For example, we are only beginning to appreciate the scope of epigenetic modifications that may be mediated by changes in metabolic flux, which could contribute to dysregulated gene expression in the context of HF. Novel modifications are now being increasingly identified, such as DNA hydroxymethylation, occurring in combination with the previously discussed PTMs on histones, as well as splice variants in the histones themselves. These modifications could all be changing in HF in complex ways and in response to comorbidities that are associated with disease progression. It is probable that systems biology approaches will be required to elucidate these complexities.
Crosstalk Between Cardiac Metabolism and Systemic Metabolism
Most studies that have examined the relationship between cardiac metabolism and HF in humans have focused on HF with reduced EF. However, HFpEF represents a major and growing clinical problem ( see also Chapter 39 ). Risk factors for HFpEF cluster with those that are associated with the metabolic syndrome such as obesity, insulin resistance, and T2D. Changes in cardiac metabolism that are associated with obesity have been reviewed extensively elsewhere. In summary, obesity is associated with increased myocardial FA utilization, decreased glucose oxidation, increased myocardial oxygen consumption, decreased cardiac efficiency, and myocardial steatosis. These changes have also been associated with or mechanistically linked in some animal models with increased myocardial fibrosis and inflammation. Given the heterogeneity of comorbidities associated with HFpEF, relatively few studies have systematically examined changes in cardiac substrate metabolism in these subjects. However, Peterson and colleagues have conducted a series of studies in individuals with obesity and the metabolic syndrome with HFpEF prior to and after bariatric surgery. Weight loss surgery was associated with improvement in systemic metabolic parameters that paralleled regression of left ventricular hypertrophy (LVH) and improvement of diastolic relaxation. In the initial studies, the only predictors of improved LV relaxation were decreased body mass index (BMI), improved insulin resistance, reduction in total MVO 2 , and LV mass. The relationship between total MVO 2 and LV relaxation was independent of BMI. However, changes in FA utilization did not predict an improvement in LV relaxation. In a follow-up study by the same group, bariatric surgery in obese women with HFpEF led to improved symptom index, regression of LVH, and improved diastolic function, which occurred in parallel with improvement in hepatic steatosis but with no change in cardiac steatosis. However, although circulating levels of ceramide and sphingolipids fell, there was no correlation between these changes and changes in LV function. Taken together, these observations suggest that systemic (noncardiac changes) might play a more important role in the pathophysiology of HFpEF than changes in cardiac metabolism per se. Potential candidate mechanisms include changes in skeletal muscle insulin sensitivity, increased skeletal muscle blood flow, decreased peripheral vascular resistance, changes in the circulating concentrations of adipokines such as adiponectin, or other novel metabolic regulators such as fibroblast growth factor-21 (FGF-21). These findings, which are consistent with clinical observations that interventions such as exercise, which might improve symptoms in HFpEF, might mediate its beneficial effects by addressing pathophysiologic mechanisms in the periphery as opposed to the heart.
Recent studies in animals have also suggested that changes in cardiac muscle per se might have profound effects on systemic metabolic homeostasis. HF is associated with increased circulating concentrations of natriuretic peptides. Studies by the Collins’ group suggest that these peptides may activate natriuretic peptide signaling pathways in brown adipose tissue that may activate the thermogenic program of brown adipocytes to increase energy expenditure. Although decreased natriuretic receptor signaling in adipose tissue has been associated with insulin resistance in subjects without HF, the relationship between this pathway and energy expenditure in HF patients remains to be determined. It will be interesting to determine, for example, if aberrant activation of thermogenesis by increased concentrations of natriuretic peptides could contribute to cardiac cachexia. Other intriguing reports in animal models have shown that manipulation of specific molecular pathways in the heart, such as the transcriptional regulator MED13, might modulate systemic glucose metabolism and insulin sensitivity via a miRNA miR-208a that is secreted from the heart.
Metabolic Remodeling and Risk for Heart Failure Development
Cardiac Hypertrophy
LVH is an independent risk factor for HF development and increases mortality by more than twofold. Pressure overload due to hypertension and aortic stenosis, and postinfarction remodeling are the most frequent causes of human LVH. Although LVH is in principle an adaptive response, this process becomes maladaptive if left untreated and may progress to cardiac dysfunction and failure. However, despite its importance, the transition from hypertrophy to HF in humans is incompletely understood. The most consistent data on energy metabolism in hypertrophied hearts are available from animals subjected to chronic pressure overload, such as surgically mediated transverse aortic constriction or spontaneously hypertensive rats. In these models, most studies report an early decrease in FAO, which is likely the consequence of decreased PPARα and also decreased PGC-1 signaling, which leads to decreased expression of CPT1 and FAO enzymes. In parallel, glucose uptake and glycolysis rates increase, while glucose oxidation may decrease and thereby impair the coupling of glycolysis to glucose oxidation. This uncoupling increases lactate production and may cause myocardial acidosis, which may contribute to contractile dysfunction. The substrate switch toward a relative increase in glucose utilization during hypertrophy is considered a reprogramming of energy metabolism to a “fetal” pattern of energy substrate metabolism and may be beneficial for cardiac energetics because glucose may represent a more “oxygen-efficient” energy substrate, in addition to other mechanisms. Increased glucose utilization during hypertrophy may result from activation of AMPK, which increases glucose uptake and stimulates glycolysis by increasing GLUT4 trafficking to the sarcolemma and by activation of phosphofructokinase. AMPK activation results from increased AMP levels, probably indicating myocardial energy starvation that is already present during the stage of compensated hypertrophy. Indeed, in animal models and in human cardiac hypertrophy, the PCr/ATP ratio is decreased, although ATP levels remain unaltered. However, after transition to HF, the PCr/ATP ratio further declines, accompanied by a relevant decrease in myocardial ATP content. In addition to altered substrate oxidation, downstream defects within mitochondria such as impaired mitochondrial respiratory capacity, ATP synthesis and OXPHOS activities have been observed during hypertrophy, a phenotype which becomes even worse after transition into cardiac failure. Although it remains a subject of debate whether a myocardial energy deficit represents the cause or consequence of hypertrophy and HF, studies in transgenic mice with increased glucose uptake or increased AMPK activity show a normalization of the PCr/ATP ratio and attenuation of HF development. These observations support the concept that energy starvation may already occur in hypertrophy or that biochemically evident but functionally as yet insignificant alterations in cardiac energy metabolism occur in hypertrophied hearts, aggravation of which following or during transition to HF ultimately contributes to cardiac dysfunction and increased risk of HF. It is likely that beneficial effects of some manipulations to increase myocardial energy utilization are more likely to succeed if flux through the entire metabolic pathway is enhanced and the coupling of substrate utilization to oxidation is optimized.
Obesity
Epidemiologic data reveal a consistent association between obesity and HF. The mechanisms linking obesity and HF are multifactorial and include increased incidence of coronary atherosclerosis, hypertension, sleep apnea and pulmonary hypertension, and volume overload. Moreover, obesity increases the risk for insulin resistance and T2D, which might also independently contribute to HF risk. Many studies in humans and animal models have also identified intrinsic changes within the heart in the context of obesity that could contribute to the increased risk of HF. These changes have been extensively reviewed and will be summarized briefly. Obesity is associated with increased myocardial FA utilization. Increased use of FA as a metabolic substrate increases myocardial oxygen consumption and reduces cardiac efficiency. Increased myocardial lipid uptake is also associated with myocardial storage of triglycerides (myocardial steatosis). Although the mechanisms linking myocardial steatosis and HF are incompletely understood, it is believed that the accumulation of lipid in the heart promotes “lipotoxicity” probably on the basis of the generation of lipid metabolites that activate signaling pathways that contribute to cellular dysfunction such as impaired autophagy or cell death. The increase in FA utilization in obesity is associated with reduced glucose uptake and oxidation, which could potentially increase the risk of myocardial injury in the context of ischemia when the dependence of metabolism on glycolysis is increased.
Studies in humans and animals with obesity have suggested that mitochondrial energy metabolism might also become impaired. Early changes that have been noted in animals with diet-induced or genetic obesity include evidence of mitochondrial uncoupling that could be related to the induction or activation of uncoupling proteins such as uncoupling protein 3 (UCP3). Increased mitochondrial ROS generation has been shown to increase the activation of UCP3 and other mitochondrial proteins such as the adenine nucleotide translocase. Activation of mitochondrial uncoupling increases mitochondrial proton leak and oxygen consumption, which has been postulated to contribute to increased rates of myocardial oxygen consumption and reduced cardiac efficiency. Obesity leads to generalized insulin resistance and hyperinsulinemia. Studies in humans and animals suggest that, in contrast to skeletal muscle in which insulin resistance is associated with reduced activation of insulin signaling intermediates, in the heart, insulin signaling might actually be increased. Insulin is known to be a potent growth factor in the heart, and hyperinsulinemic activation of insulin signaling might contribute to LVH. In some animal models, protracted high-fat feeding may lead to spontaneous ventricular dysfunction that is associated with altered signaling via forkhead transcription factors, which is a downstream mediator of insulin signaling. Animal studies have also suggested a crosstalk between myocardial insulin signaling and adrenergic signaling pathways that promote myocardial fibrosis. Obesity and insulin resistance might also contribute to myocardial pathology by increasing inflammatory signaling.
Diabetes Mellitus
Diabetes mellitus increases the risk of HF. The burden of HF in diabetes is largely borne by individuals with T2D, which accounts for greater than 95% of all diabetes. Epidemiologic studies reveal clear evidence of subclinical myocardial injury, as evidenced by troponin leak, in individuals with no clinical evidence of HF but who have diabetes or are at high risk for developing T2D. In addition, many asymptomatic patients with T2D exhibit evidence of impaired diastolic relaxation that can be detected by echocardiography, particularly when coupled with speckle tracking. The increased risk of HF in diabetes has been termed diabetic cardiomyopathy. Mechanisms for diabetic cardiomyopathy have been extensively reviewed. There is overlap between the mechanisms described previously linking HF and obesity and those that link diabetes and HF. However, it is very likely that given additional systemic metabolic abnormalities such as hyperglycemia and elevated circulating concentrations of FA and inflammatory cytokines, the myocardial maladaptations in diabetes are more severe than those described in obesity. For example, diabetes mellitus is invariably associated with impaired mitochondrial OXPHOS capacity that exacerbates mitochondrial energetic impairment and mitochondrial uncoupling. In addition, oxidative stress is likely to be more severe in diabetes relative to individuals who are obese. Hyperglycemia also activates signaling mechanisms described elsewhere in this chapter, such as those that are downstream of the hexosamine biosynthetic signaling pathway. Other pathways linked to glucotoxicity include accumulation of advanced glycation end products, which have been implicated in the pathogenesis of diabetes-associated myocardial fibrosis. Impaired excitation-contraction (E-C) coupling secondary to impaired function of the sarcoplasmic reticulum (SR) has also been described. It is noteworthy that simply normalizing blood glucose might not be sufficient to mitigate the risk of HF in diabetes. Indeed, many therapeutic glucose-lowering agents have been suggested to increase the risk of HF hospitalizations, whereas others do not. Thus a consideration of cardiovascular outcomes has now become an important facet in the approval of new glucose-lowering agents and an important consideration in the choice of agent when treating individuals at high risk for cardiovascular complications. Recent trials have revealed a reproducible effect of a relatively new class of antidiabetic agents, SGLT2 inhibitors, in reducing not only cardiovascular mortality but also the prevalence of HF and hospitalization for HF. Although there has been much speculation regarding the mechanisms for the cardioprotection such as increasing circulating ketones, volume contraction, and blood pressure lowering, the basis for the beneficial impact of SGLT2 inhibitors on HF and cardiovascular (CV) mortality is not understood.
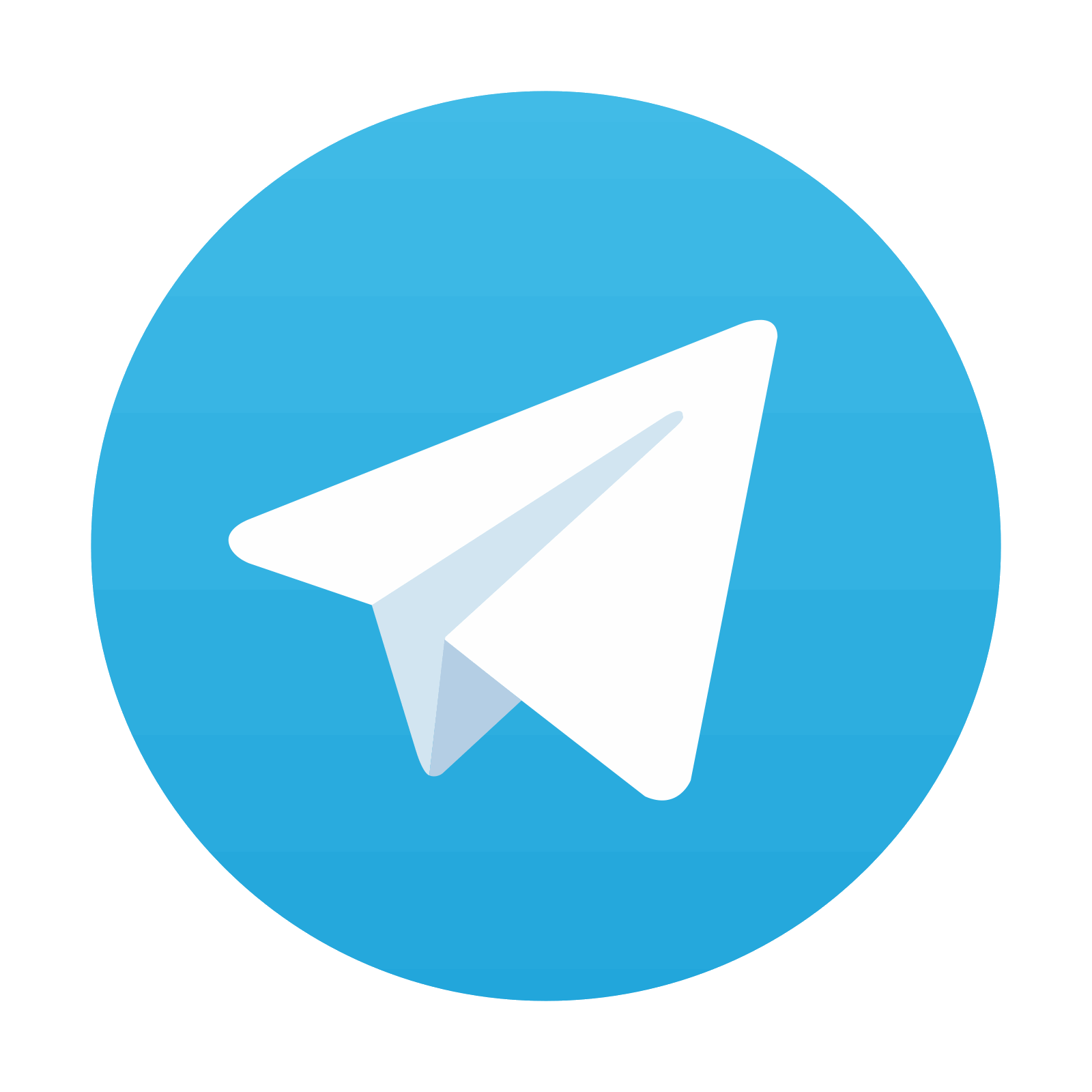
Stay updated, free articles. Join our Telegram channel
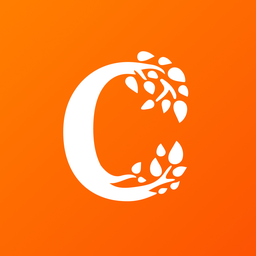
Full access? Get Clinical Tree
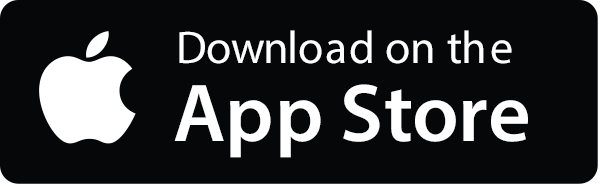
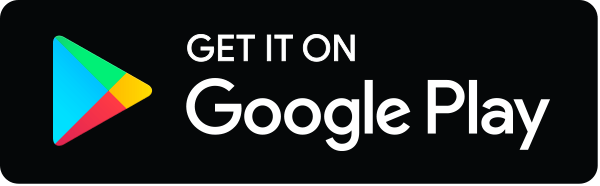