Stem Cell Therapy for Ischemic Heart Disease
Marc S. Penn
Ischemic heart disease remains the leading cause of congestive heart failure (CHF). The prevalence of CHF has increased dramatically over the preceding decade, with now more than 10% of the United States population older than 65 years of age carrying the diagnosis. Based on current trends, the problem is predicted to increase to greater than 6 million people by the year 2030 (1).
The mechanisms are still under investigation (2,3,4), but the development of CHF following myocardial infarction (MI) is more than just the loss of contractile tissue; it is also partly determined by the ventricular remodeling that occurs in response to myocardial necrosis (5,6). The inflammatory response to myocardial necrosis leads to infarct expansion, dilatation of the left ventricular (LV) cavity, and replacement of cardiomyocytes with fibrous tissue (2,6). Currently available therapies to alter the remodeling process and the progression to CHF remain limited, and death rates from CHF continue to rise. Furthermore, although cardiac transplantation is a definitive therapy for severe end-stage CHF, it remains limited in number and in some instances simply trades one set of symptoms for another. Given the epidemic proportions that the number of patients with CHF is reaching, coupled with the enormous burden that these patients bring to the health care system, there is significant interest in optimizing or regenerating myocardial tissue following the ischemic injury. One of the approaches under intense investigation is cell therapy. The goal of this chapter is to summarize the literature on cell therapy for the treatment of LV dysfunction and to attempt to give a clinical perspective on the potential of this exciting emerging therapeutic strategy.
Approaches to Cell Therapy
The field of cell transplantation for the treatment of left ventricular dysfunction following ischemic injury is broad (7). Theoretically, optimal cell therapy will use cell types that possess the capacity to incorporate into the recipient myocardium and that will be able to survive, mature, and electromechanically couple to each other and to native cardiac myocytes.
In its simplest form, differentiated cell transplantation (Fig. 101.1A), the goal is to transplant autologous cells that are differentiated, or committed to differentiate into specific cells, into areas of myocardial scar, with the goal to replace areas of scar tissue with living viable cells. In perhaps its most futuristic form, stem cell mobilization (Fig. 101.1B), cell therapy involves the pharmacologic mobilization of a patient’s own bone marrow cells into the blood stream, homing of these stem cells to engraft into areas of myocardial damage, and then directing the engrafted stem cells to differentiate into cardiac myocytes and vascular structures that fully integrate with the native myocardium. An in-between strategy, stem cell transplantation, (Fig. 101.1C), is the removal of stem cells from the bone marrow of the patient, and then injection of the whole bone marrow or a selected population of bone marrow–derived stem cells into the infarct zone. Whereas each of these strategies has its own unique technological and clinical challenges, all share a number of important questions: (i) What is the optimal cell type or class of cells for each strategy? (ii) When is the earliest time following acute MI that the cells would be available for transplantation? (iii) Balancing safety and efficacy, when is the optimal time following acute MI for initiation of cell therapy? (iv) What is the mode of efficacy for a specific strategy (prevention of remodeling or regeneration of contractile tissue)? and (v) Do the engrafted/transplanted cells represent an arrhythmogenic risk? Both bench animal and clinical research have significantly expanded our knowledge, but no cell-based strategy to date has come close to achieving all these parameters. That said, exciting therapeutic strategies are beginning to emerge from the animal into the phase I clinical trial arena (8,9,10,11).
Differentiated Cell Transplantation
As mentioned above, the goal of differentiated cell transplantation is the substitution of scarred myocardium with living viable cells ultimately leading to overall improvement of myocardial function. A number of cell types, including smooth muscle cells (12) and skeletal myoblasts (13), have been studied as potential candidates for differentiated cell transplantation. The
value of these cell types is born out of their accessibility and their ability to be expanded in vitro before transplantation.
value of these cell types is born out of their accessibility and their ability to be expanded in vitro before transplantation.
The majority of the animal work in this field focused on the use of skeletal myoblasts and, specifically, on skeletal myoblast (SKMB) transplantation. In contrast to cardiac myocytes, SKMBs maintain their ability to regenerate and, during periods of stress, to proliferate and differentiate into myotubes, eventually forming new muscle fibers capable of contraction. In addition, skeletal muscle is much more resistant to ischemia than is cardiac muscle. Skeletal muscle can withstand many hours of ischemia without becoming irreversibly injured, compared with irreversible injury occurring within 20 minutes in cardiac muscle (14).
Although these are desirable properties for cell therapy, the timing of delivering SKMBs before pathological LV remodeling following MI in clinical populations is problematic. It takes at least 3 to 4 weeks between skeletal muscle biopsy and the availability of a sufficient number of SKMBs for transplantation in humans. Thus, if the main benefit of skeletal myoblast transplantation is to attenuate left ventricular remodeling, then unless patients who will benefit can be identified very early after MI and/or an off-the-shelf cell that is not immunogenic will be available at the time of MI, it is debatable as to whether SKMB transplantation alone will offer significant clinical benefit.
SKMB therapy is the most studied cell-based therapy in clinical populations to date. Because of the above limitations in the timing of SKMB therapy to patients with recent MI, clinical studies have focused on patients with ischemic cardiomyopathy. The potential of skeletal myoblast engraftment and survival in humans with end-stage ischemic cardiomyopathy was recently demonstrated (15,16,17). In the U.S. study, transplantation with 300 million skeletal myoblasts was performed at the time of LV device placement in patients with end-stage CHF due to ischemic cardiomyopathy (15). At the time of cardiac transplantation, the explanted hearts were assessed for the presence of skeletal myoblasts. In patients 3, 4, and 6 months after skeletal myoblast transplantation, immunohistochemistry revealed the presence of skeletal myoblasts. In the one patient who received 2.2 million cells, perhaps not surprisingly, no evidence of skeletal myoblast engraftment was found. Importantly, at least for a dose up to 300 million cells, skeletal myoblast transplantation did not appear to be arrhythmogenic. However, studies with higher doses of skeletal myoblasts have suggested an increase in ventricular tachycardia in the days following transplantation. Although this is obviously an important concern, recent studies would indicate that the majority of patients considered for this therapy would be eligible for and may benefit from implantation of internal cardiac defibrillators (18,19).
That said, the mechanism of functional LV improvement with SKMB transplantation is not clear and is still under investigation. Transplanted skeletal myoblasts do not result in the development of new cardiac myocytes (20), and the transplanted cells do not electromechanically integrate the native myocardium (21). Furthermore, increases in LV ejection fraction observed with the introduction of skeletal myoblasts into the infarct zone are modest (22,23,24). However, the combined improvement in systolic function with the improvements in diastolic function that have been demonstrated ultimately appear
to lead to overall improved cardiac performance (25,26). Despite these unknowns, there is real hope that skeletal myoblasts transplantation will benefit clinical populations, even at times remote from MI (27), and it is now under investigation in phase I and II clinical trials (27).
to lead to overall improved cardiac performance (25,26). Despite these unknowns, there is real hope that skeletal myoblasts transplantation will benefit clinical populations, even at times remote from MI (27), and it is now under investigation in phase I and II clinical trials (27).
Finally, the full potential of differentiated cell transplantation may not have been realized yet. The time during which skeletal myoblasts are expanded in culture before transplantation offers a unique opportunity to engineer these cells to express soluble factors (e.g., vascular endothelial growth factor, VEGF) before transplantation. The injection of skeletal myoblasts that were transfected with VEGF-165 encoding cDNA into the rat myocardium at the time of left anterior descending (LAD) artery ligation resulted in increased vascular density within the infarct zone and decreased infarct size (28). Furthermore, recent data from our group suggests that transfection of skeletal myoblasts with adenovirus encoding VEGF-165 the night before transplantation results in significantly greater recovery of left ventricular function in a rat model of ischemic cardiomyopathy compared with the direct injection into the myocardium with the same amount of adenovirus (29). Thus, it is possible that viral vectors expressing a gene product of interest that are presently undergoing clinical trials could be combined with skeletal myoblast transplantation and yield a greater clinical benefit (30,31).
Myocardial Regeneration
Whereas differentiated cell transplantation offers the possibility of replacing dead or dying scar tissue with living cells, stem cell therapy is developing into a new approach that may be capable of regenerating myocardial tissue and function. The excitement surrounding the use of stem cells is based on the unique biological properties of these cells and their capacity to self-renew and regenerate tissue and organ systems (32). It was fewer than 5 years ago that Orlic et al. demonstrated that the direct injection into the infarct border zone of specific populations of bone marrow–derived stem cells within hours of LAD ligation had the potential to regenerate cardiac myocytes and blood vessels within the infarct zone (33). In their experiment, they injected CD117+ (c-kit positive, a marker of hematopoietic stem cells) bone marrow–derived stem cells from green fluorescent protein (GFP)-positive mice into the periinfarct zone 2 hours after LAD ligation. Nine days later, they showed that there were GFP-positive cells in the infarct zone and that many of these cells also stained positive for cardiac myosin. The fact that GFP-positive cells were cardiac myosin–positive suggests that the injected stem cells migrated into the infarct zone and then differentiated into cardiac myocytes. This study went on to show that the injection of CD117– stem cells did not result in regeneration of myocardial tissue.
Simultaneously, a study was published demonstrating that the intravenous infusion of human angioblasts (CD34+) into nude rats 48 hours after anterior wall MI led to engraftment of these angioblasts into the infarct zone and nearly 70% recovery of cardiac index 6 weeks later (34) despite no regeneration of cardiac myocytes within the infarct zone. This study solidified the concept that stem cells home to the injured myocardium during the periinfarct period and that developing strategies to increase stem cell engraftment and vasculogenesis within the infarct zone may lead to improvement in the cardiac function, even in the absence of regeneration of cardiac myocytes.
Since these landmark studies, many studies have been published that argue against the concept that hematopoietic stem cells have the ability to differentiate into cardiac myocytes (35,36). Although controversy still exists regarding whether these early experiments of Orlic et al. actually resulted in regeneration of cardiac myocytes, it is critical to note that no controversy exists as to whether myocardial function was improved by the delivery of hematopoietic stem cells to the heart at the time of MI.
Since these early studies, a significant body of literature has rewritten the once strongly held belief that the heart cannot repair itself. We have learned that following MI in humans, there is a transient mobilization of stem cells (37). Human female hearts transplanted into men were found to have cardiac myocytes and vascular structures that stained positive for the Y-chromosome, suggesting that these cells originated from the stem cells from the transplant recipient (38,39,40). Finally, immunohistochemical studies of human hearts from patients who expired within days following MI demonstrated cardiac myocytes that had divided (41). Because cardiac myocytes maybe unlikely to divide, it is possible that these dividing cardiac myocytes were recently engrafted and differentiating bone marrow–derived stem cells. Unfortunately, these studies demonstrate that stem cell engraftment and differentiation into cardiac myocytes is an infrequent event (0.02% cardiac myocytes, 3.3% endothelial cells [42]). However, these studies do suggest that the normal physiologic response to myocardial injury is mobilization of stem cells, homing of these cells to the damaged myocardium, and differentiation of at least some of these stem cells into cardiac myocytes. Furthermore, if this natural repair mechanism can be potentiated, clinically meaningful myocardial regeneration may be achievable. That stem cells can home to injured myocardium suggest the possibility that transplantation of stem cells may not be necessary, but rather, mobilization of endogenous stem cells could lead to the same effect.
The early studies using stem cells to recover cardiac function following MI focused on hematopoietic stem cells. Since these studies, several other potential sources as well as types of adult stem cells have been identified. Each of these cell types has distinct advantages with respect to its widespread availability for clinical applications, as well as its differing potential to truly regenerate cell types that are present in the myocardium.
Mesenchymal Stem Cells and Multipotent Adult Progenitor Cells
Stromal progenitor cells make up less than 0.05% of adult bone marrow. Mesenchymal stem cells (MSCs) and multipotent adult progenitor cells (MAPCs) form subpopulations of bone marrow stromal progenitor cells that maintain the ability to differentiate along multiple lineages (43,44,45). MSCs can be immunoselected via cell surface CD45 negativity and cultured through as many as 40 population doublings before attaining senescence (46). 5-Azacytidine, a DNA demethylating agent, can induce MSCs to differentiate into cardiac myocytes in vitro. Using rat and swine infarction models, MSCs have been shown to have the ability to differentiate into cardiac myocytes (47). Moreover, hematopoietic stem cell (HSC) differentiation into blood vessel cells coordinated with MSC differentiation into cardiac myocytes results in improved regional perfusion and wall motion, increased scar thickness, and enhanced global heart function. Non-5-azacytidine treated MSCs directly injected into the infarct zone of swine hearts engrafted in the host myocardium and demonstrated expression of muscle-specific, but not cardiac-specific, proteins. In these studies, engraftment may have helped to attenuate contractile dysfunction and pathologic thinning (48).
MAPCs are able to differentiate into endothelial, epithelial, and mesenchymal cell types (49,50). They can be expanded in culture for more than 80 population doublings and still maintain their pluripotency by differentiating into most somatic cell types (49). MAPC identification techniques include negative staining for several surface markers: CD34, CD44,
CD45, CD117, and major histocompatibility complex class I and II after several weeks in culture under strict conditions. This poses a problem because at present, MAPCs cannot be selected early based on specific phenotypic markers (49). Other multipotent adult stem cells have recently been described that appear capable of differentiating into cardiac myocytes in vivo (51). Whether these two multipotent stem cells exist in vivo or are the result of serial cell passaging in culture remains to be seen. However, the demonstration that multipotent cells can be significantly expanded bodes well for the development of future therapies.
CD45, CD117, and major histocompatibility complex class I and II after several weeks in culture under strict conditions. This poses a problem because at present, MAPCs cannot be selected early based on specific phenotypic markers (49). Other multipotent adult stem cells have recently been described that appear capable of differentiating into cardiac myocytes in vivo (51). Whether these two multipotent stem cells exist in vivo or are the result of serial cell passaging in culture remains to be seen. However, the demonstration that multipotent cells can be significantly expanded bodes well for the development of future therapies.
Umbilical Cord Blood Stem Cells
The stem cell compartment in cord blood is less mature than that of adult bone marrow. HSCs are less abundant in bone marrow than in cord blood, where they demonstrate greater proliferative potential associated with longer telomeres and an extended life span (52,53,54). In addition to these benefits, cord blood is regularly harvested with little if any risk to the donor, is abundantly accessible, and associated infectious agents are rare (e.g., cytomegalovirus) (55). Such characteristics indicate that umbilical cord–derived HSCs hold promise and may advance cellular cardiomyoplasty. Importantly, umbilical cord blood stem cells offer an abundant source of stem cells free of invasive techniques and ethical concerns.
More recently, MSC populations derived from umbilical cord blood have been described (56,57). These cells may offer the benefit of cardiac myogenesis, rather than just angiogenesis (58), and are a noninvasive, noncontroversial source of stem cells. The circulatory capacity of the HSC is evidenced in adult transplantation studies, umbilical cord blood (59), and peripheral blood under unique conditions. Despite the lack of direct evidence for the presence of MSCs in the blood, the proof of their circulatory and homing capacity is observed in the engraftment of MSCs posttransplantation (60). In one study, the ability of umbilical cord blood to generate cells with the characteristics of mesenchymal progenitor cells in culture was examined. These studies showed that cord blood is richer in mesenchymal progenitors, similar to hematopoietic progenitors, preterm as compared with term (61).
Unrestricted somatic stem cells (USSCs) appear to be an intrinsically pluripotent, CD45–, HLA class II null population from human cord blood. This rare population can be expanded to 1015 cells while continuing to maintain its pluripotency and is grown in adherent cultures. USSCs demonstrate intrinsic and directable potential to develop into mesodermal, endodermal, and ectodermal cell fates and are replete with in vitro proliferative capacity but do not spontaneously differentiate. Many months after human USSC transplantation in sheep hearts, substantial numbers of human cardiomyocytes in both atria and ventricles were identified (62).
Embryonic Stem Cells
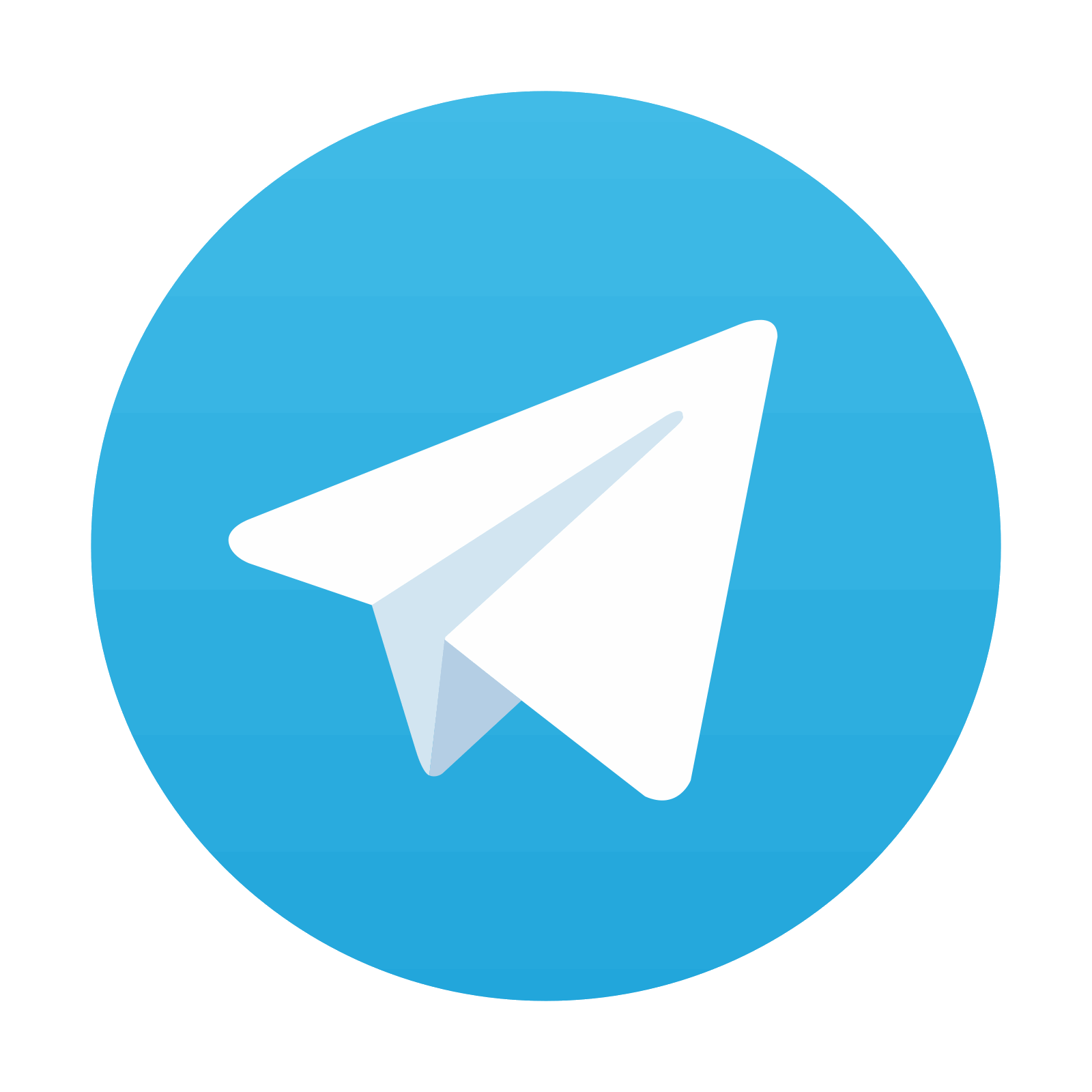
Stay updated, free articles. Join our Telegram channel
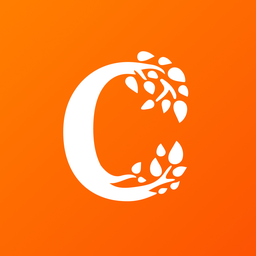
Full access? Get Clinical Tree
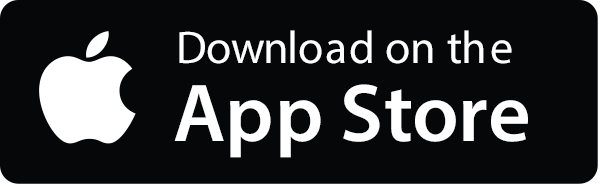
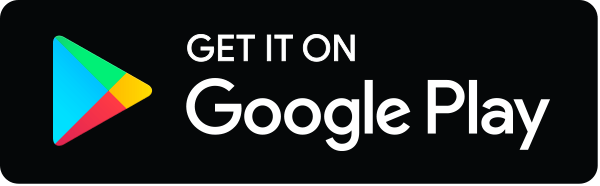