Characteristics of sleep at high altitude Improving sleep at high altitude Traveling to high altitude with known obstructive sleep apnea Everyone who has been to high altitude knows that sleeping is initially impaired. This ubiquitous problem affects the skier or trekker who sleeps at altitudes of 2500–3000 m, as well as the well-acclimatized climber who spends time as high as 8000 m. Common complaints include difficulty falling asleep, frequent awakenings, and persistent feelings of fatigue upon awakening in the morning. Vivid dreams and racing thoughts are other common features for many people. Underlying these subjective complaints are important changes in sleep physiology, including changes in sleep architecture and the control of breathing. Alterations in the latter frequently lead to periodic breathing, a major contributor to altered sleep quality at high altitude that has been recognized as a problem since the 19th century. This common problem, which can cause severe degrees of hypoxemia following apneic periods at extreme altitudes (West et al. 1986), may be one of the factors that influences tolerance to very great altitudes and sheds light on the control of breathing under these special conditions. This chapter considers the physiology of sleep at high altitude in greater detail, including sleep architecture, control of breathing, and the periodic breathing phenomenon noted above. Consideration is also given to pharmacologic and nonpharmacologic measures to improve sleep quality in this environment, as well as changes that may occur in patients with sleep-disordered breathing at sea level who travel to higher elevations. The material considered here overlaps somewhat with that of Chapter 9, as control of ventilation has a large influence on breathing patterns during sleep Dating back many years, there have been a number of anecdotal references to the poor quality of sleep at high altitude. Barcroft gave a particularly colorful description when he recounted his experiences during the glass chamber experiment carried out at Cambridge, UK (Barcroft et al. 1920). On that occasion he spent six days in a closed chamber in which the concentration of oxygen was regulated so that the initial equivalent altitude was 3048 m and the final altitude 4877 m. He wrote: Barcroft’s impression that, despite a normal sleep time, sleep is less refreshing than normal due to the increased frequency of awakenings has proven to be accurate over time as more recent studies have subsequently confirmed this finding (Reite et al. 1975; Zieliński et al. 2000). Various references to the uneven pattern of breathing during sleep at high altitude were made during the 19th century. One was by the eminent English physicist Tyndall, one of the most ardent Alpine mountaineers in the middle of the century about whom Paul Bert once commented, “every year sees him planting his alpenstock on some new summit” (Bert 1878). During Tyndall’s first ascent of Mont Blanc in 1857, he became very fatigued and laid down to rest. He subsequently wrote: Another early comment on periodic breathing was made by Egli-Sinclair (1893) in an article on mountain sickness. He noted that, at an altitude of 4400 m, respiration had the Stokes character, that is, it seemed regular during a certain time, after which a few rapid and profound breaths were drawn, a total suspension of a few seconds then following. Here he was referring to the Irish physician, William Stokes, who described the pattern of breathing, which consists in the occurrence of a series of inspirations, increasing to a maximum and then declining in force and length until a state of apparent apnea is established (Stokes 1854). Another Irish physician, John Cheyne, had described the same pattern in 1818 (Cheyne 1818) and so the breathing pattern became known as Cheyne–Stokes breathing, although Ward (1973) pointed out that John Hunter had given a lucid and succinct description of the same condition in 1781 (Hunter 1781). The first extensive studies of periodic breathing at high altitude were made by Angelo Mosso, Professor of Physiology at the University of Turin, Italy, who, as mentioned earlier, was one of the first people to use the Capanna Regina Margherita on the Monte Rosa (4559 m) for scientific work. He measured the breathing movements by means of a lever that rested on the chest. An example of one of his measurements on his brother, Ugolino Mosso, showed that periods of apnea lasted about 12 seconds. Follow-up observations revealed that the waxing and waning of breathing movements were interspaced by the shorter periods of apnea (Mosso 1898). Sleep can be defined as a state of unconsciousness from which the subject can be aroused by sensory or other stimuli. As such it can be distinguished from deep anesthesia and disease states that cause coma, though these have some features in common with true sleep. Two major types of sleep are recognized. This is often called non-REM or NREM (nonrapid eye movement) sleep, or sometimes normal sleep. It is characterized by decreased activity of the reticular activating system, and is called slow wave sleep (SWS) because of the predominance of slow delta waves in the electroencephalogram (EEG). These slow waves have a high voltage and occur at a rate of one or two second−1. In the early stages of sleep, the alpha rhythm (8–13 Hz), which is always present during wakefulness, becomes more obvious. In addition, sleep spindles (14–16 Hz) may appear. These features can be used to divide SWS into four stages (I–IV). The delta waves probably originate in the cortex of the brain when it is not driven from below because of the reduced level of activity of the reticular activating system. SWS is dreamless, very restful, and associated with a decreased peripheral vascular tone, blood pressure, respiratory rate, and basal metabolic rate. This is called REM sleep because, although the eyes remain closed, there are rapid horizontal eye movements. In a normal night of sleep, bouts of REM sleep lasting five to 20 minutes usually appear on average about every 90 minutes. The first such period occurs 80–100 minutes after the subject falls asleep and then more frequently through the night. The EEG tracing resembles the waking state, but the person is actually more difficult to arouse than during NREM sleep. REM sleep is usually associated with active dreaming. Muscle tone throughout the body is greatly depressed, but there may be occasional muscle twitching and limb jerking. The heart rate and respiration usually become irregular. Thus, in this type of sleep, the brain is quite active but the activity is not channeled in the proper direction for the person to be aware of his or her surroundings. In general, minute ventilation is depressed during sleep compared to the awake state, as are the ventilatory responses to hypoxemia and carbon dioxide. The hypercapnic ventilatory response appears to be reduced in both NREM and REM sleep (Bulow 1963; Douglas et al. 1982a), but there is more uncertainty about the hypoxic ventilatory response; some studies indicate that it is increased in NREM sleep (Pappenheimer 1977; Phillipson et al. 1978), while other studies suggest it is decreased in all sleep stages including REM sleep (Berthon-Jones and Sullivan 1982; Douglas et al. 1982a, 1982b). While these observed changes in minute ventilation and the ventilatory responses to chemical stimuli are often attributed to loss of the wakefulness drive to breathe during sleep (Douglas 2000; Orem and Kubin 2000) important changes in neurochemical regulation of breathing, including enhanced gamma aminobutyric acid (GABA) activity, also play a significant role (Joseph et al. 2002). The decrease in minute ventilation is also due in part to changes in upper airway patency during sleep that occur as a result of both mechanical factors, as well as changes in neurochemical control of pharyngeal motor neurons responsible for maintaining upper airway tone (Dempsey et al. 2010). By causing sleep deprivation or fragmentation, alterations in the normal sleep patterns described above impair central nervous system function, with the higher brain functions being the most susceptible. There are similarities between the behavior of sleep-deprived individuals and people at high altitude whose brains are affected by hypoxia. In both instances, mental activities that are “mechanical” in nature, such as tabulating a set of data, can be accurately accomplished, whereas activities that require problem solving and initiative are seriously affected (Chapter 12). It may be that some of the impairment of central nervous system (CNS) function in individuals living at high altitude can be ascribed to the poor quality of sleep, but the direct effects of hypoxia on the brain also clearly play a role. In this section, we consider how sleep is affected by hypobaric hypoxia with the discussion focused largely on changes reported in adults. The limited information available about sleep in children at high altitude is considered in Chapter 26. People at high altitude often report that they wake more frequently during the night than at sea level. This phenomenon has been confirmed in several careful studies (Reite et al. 1975; Salvaggio et al. 1998; Weil et al. 1978; Zieliński et al. 2000) using continuous recordings of the EEG, electromyography (EMG), and eye movements, during which arousal was recognized by the occurrence of EMG activation, eye movements, and alpha wave activity on the EEG. In one study, for example, an average of 36 arousals per night occurred at an altitude of 4300 m compared with 20 at sea level (Weil et al. 1978). An example of frequent arousals is shown in Figure 17.1. Administration of the drug acetazolamide, which is known to stimulate ventilation at high altitude and, importantly, stabilize breathing during sleep, reduced the frequency of arousals. Figure 17.1Example of the change in sleep architecture in a subject measured at sea level (upper tracing) and on the first night at an altitude of 4300 m (lower tracing). Time is on the horizontal axis and sleep stages are shown on the vertical axis. A, awake; R, REM; D, stage I; 2, stage II; 3–4, stages III and IV. At altitude, there was greatly increased sleep fragmentation and a reduction in slow wave sleep. (From Reite et al. 1975.) Some investigators believe that the arousals are caused in some way by periodic breathing and there is some evidence that arousals are more frequent when the strength of periodic breathing is high. It is easy to imagine that the strenuous muscular activity required to generate large breaths after a prolonged period of apnea could contribute to an arousal. A common nightmare at high altitude is that the tent has been covered with snow by an avalanche and the subject wakes violently feeling suffocated and very short of breath. This may be associated with the air hunger caused by long apneic periods during periodic breathing. Periodic breathing cannot be solely responsible for the increased frequency of arousals at high altitude, however, as arousals also occur in individuals who do not develop periodic breathing (Reite et al. 1975; Wickramasinghe and Anholm 1999). Multiple EEG studies have provided objective evidence of changes in sleep architecture at high altitude that support subjective conclusions that sleep at high altitude is often of poor quality, and not as refreshing as sleep at sea level. One of the earliest studies in this regard was performed by Joern et al. (1970), who evaluated changes in sleep architecture near the South Pole where the barometric pressure is reduced because of the actual altitude and also the very high latitude (Chapter 2). They reported a near absence of sleep stages III and IV coupled with an approximately 50% reduction in REM sleep. Although the light-dark cycle was atypical compared to other sleep settings, their findings have been confirmed at more moderate latitudes. Several years later, Reite et al. (1975) studied sleep patterns in subjects following a rapid ascent to Pikes Peak (4300 m). They found a similar shift from deeper to lighter sleep stages and a great reduction in REM sleep. Periodic breathing was common but disappeared during REM sleep. The changes in the pattern of sleep and respiration were greatest on the first night at high altitude and then declined thereafter. Subsequent studies have generally confirmed the findings that time spent in light sleep (stages I and II of NREM) is increased, while the time spent in deep sleep (stages III and IV of NREM) is decreased. The data regarding the time spent in REM sleep have been conflicting, however, with some studies reporting that it is virtually abolished (Megirian et al. 1980; Pappenheimer 1977) and other studies reporting either a decrease (Anholm et al. 1992; Goldenberg et al. 1992) or no change in the time spent in this stage (Johnson et al. 2010a; Normand et al. 1990; Zieliński et al. 2000). Few studies have examined the impact of high altitude on the hypercapnic and hypoxic ventilatory responses during sleep. In one of the few studies on this issue, White et al. (1987) monitored hypercapnic ventilatory responses in six healthy men on nights 1, 4, and 7 at 4300 m and found that the response was diminished to similar degrees seen at sea level during both NREM and REM sleep. They also observed that minute ventilation fell from wakefulness to NREM and REM sleep with the bulk of the decrease being attributable to a decrease in tidal volume. Minute ventilation did increase over time at altitude, largely due to increases in respiratory rate. The common pattern of periodic breathing has now been confirmed in many studies carried out at various altitudes from sea level up to 8050 m (Berssenbrugge et al. 1983; Douglas and Haldane 1909; Douglas et al. 1913; Johnson et al. 2010a; Lahiri et al. 1983; Martin et al. 1978). A typical pattern recorded at an altitude of 6300 m (PB 351 mmHg) in a well-acclimatized lowlander is shown in Figure 17.2 (West et al. 1986). Note that the tidal volume waxed and waned during each burst of breathing, with apneic periods of about eight seconds. Arterial oxygen saturation (SaO2) as measured by ear oximeter fluctuated with the same frequency as the periodic breathing, although there was a phase difference in these changes with the highest (SaO2) (inverted scale) occurring at approximately the end of the apneic period. These fluctuations in SaO2 can likely be accounted for by being on the steep part of the oxygen dissociation curve and by the related circulation time from the lung capillaries to the ear where the oxygen saturation was measured. Heart rate was measured from the electrocardiogram (ECG) and showed marked fluctuations with the same frequency as the periodic breathing. Likely reflective of some cardiopulmonary baroreflex mechanisms, the highest heart rate appeared at the end of the burst of ventilation. Figure 17.2Example of periodic breathing at altitude of 6300 m (PB 351 mmHg). (From West et al. 1986.) Beyond the fact that periodic breathing is common among lowlanders who ascend to high altitude, there are several important features of this phenomenon. The first feature is that it persists for a considerable period of time at high altitude. In the study from which Figure 17.2 is taken (West et al. 1986), all eight subjects showed obvious periodic breathing during the several weeks over which the measurements were made at 6300 m. Similarly, Bloch et al. (2010) studied nocturnal breathing patterns in 34 mountaineers during ascent and descent from Muztagh Ata (7546 m) and found not only a persistence of periodic breathing over a several-week period but also that climbers spent a greater time in periodic breathing upon returning to base camp at the end of their climb when compared to their stay at base camp during the ascent. Despite persistence of periodic breathing over time at high altitude, overall sleep quality seems to improve with acclimatization (Nussbaumer-Ochsner et al. 2012a). As summarized in Figure 17.3, the extent of periodic breathing during sleep at altitude intensifies rather than improves with duration and severity of exposure. Figure 17.3Summary of the key studies to date that have measured apnea–hypopnea index (AHI) at varying altitudes. Note that the AHI intensifies with progressive ascent to altitude. Each number refers to a different study where: (1) Lahiri et al. (1983); (2) West et al. (1986); (3) Normand et al. (1990); (4) Salvaggio et al. (1998); (5) Kohler et al. (2008); (6) Bloch et al. (2010); (7) Insalaco et al. (2012); (8) Andrews et al. (2012); (9) Masuyama et al. (1989); (10) Lombardi et al. (2013); (11) Rey de Castro et al. Liendo (2017)); (12) Tellez et al. (2016); (13) Pham et al. (2017a); (14) Patrician et al. (2018); (15) Netzer and Strohl (1999); (16) Burgess et al. (2008); (17) Lombardi et al. (2013); (18) Burgess et al. (2013); (19) Rexhaj et al. (2016); (20) Rey de Castro et al. (2017); (21) Hughes et al. (2017); (22) Tseng et al. (2015); (23) Latshang et al. (2017); (24) Castellanos-Ramírez et al. (2018); (25) Anderson et al. (2015); (26) Pham et al. (2017b); (27) Tellez et al. (2016); (28) Pham et al. (2017); (29) Patrician et al. (2018). The putative mechanisms for the persistence or increase in periodic breathing at high elevations is not clear but is discussed further below and reviewed in detail elsewhere (Ainslie and Burgess 2017; Ainslie et al. 2013). Regardless, since the increase in apnea–hypopnea index (AHI) with duration and extent is generally reflected in less AMS, and because periodic breathing may elevate rather than reduce mean SaO2 during sleep, this may represent an adaptive rather than maladaptive response (see later discussion). Another important feature is that the percentage of time occupied by periodic breathing increases with altitude. For example, Waggener et al. (1984) reported that periodic breathing with apnea occupied 24% of the time at 2440 m and that the percentage increased to 40% at 4270 m. This increase in proportion of time is consistent with a theoretical model discussed below (Khoo et al. 1982) and has been documented in other studies (Ainslie et al. 2013; Bloch et al. 2010; Insalaco et al. 2012; Johnson et al. 2010a). Multiple studies have shown that periodic breathing is very common during NREM sleep at high altitude and may even occur during the drowsy period that precedes sleep onset (Berssenbrugge et al. 1983; Reite et al. 1975). It typically does not occur during REM sleep at moderate altitudes, although periodicity may still occur during REM sleep at high elevations, a phenomenon that has been reviewed elsewhere (Wickramasinghe and Anholm 1999; Ainslie et al. 2013). Coincidentally, at least when examined at sea level, the apneic threshold (i.e., the transient reduction in PaCO2 that causes an apnea) that resides within 2–5 mmHg PaCO2 below normal awake levels in healthy subjects in NREM is not readily demonstrable in phasic REM (Xi et al. 1993). The ventilatory response above eupnea to added CO2 is also blunted in NREM (versus wakefulness) in part due to a truly reduced CO2 chemoreceptor sensitivity (Guyenet et al. 2018); there is also a loss of tonic neural motor input to pharyngeal dilator muscles resulting in increased upper airway resistance (Henke et al. 1990). Transient arousal at apnea termination temporarily restores this tonic input and reduces airway resistance, thereby contributing to ventilatory overshoot prior to sleep restoration. Similarly, in REM sleep ventilatory responsiveness to CO2 above eupnea is reduced in slope and shows an almost random tidal volume and/or diaphragmatic EMG responses to increasing PaCO2 (with or without coincident airway occlusion), rather than an orderly dose-response as in quiet wakefulness or NREM sleep (Smith et al. 1997). The extrapolation of these carefully conducted laboratory studies at sea level to high altitude needs to be established in future research. Early evidence indicated that cycle length of the apnea decreases with increasing altitude (Waggener et al. 1984); however, data from the American Medical Research Expedition to Everest (AMREE) reported a mean cycle period of only 20.5 seconds, a value similar to that measured in a companion study at 5400 m (Lahiri et al. 1983). Consistent with this latter observation, a summary of the studies conducted at high altitude illustrate that cycle lengths remain somewhat stable at high altitude (Figure 17.4). Figure 17.4Relationship between altitude and apnea-hypopnea duration. Each number refers to a different study where: (1) Nicholson et al. (1988); (2) Anholm et al. (1992); (3) Eichenberger et al. (1996); (4) Fischer et al. (2004); (5) Burgess et al. (2004); (6) Kohler et al. (2008); (7) Mees and de la Chaux (2009); (8) Bloch et al. (2010); (9) Clarenbach et al. (2012); (10) Nussbaumer-Ochsner et al. (2012a); (11) Andrews et al. (2012); (12) Nussbaumer-Ochsner et al. (2010); (13) White et al. (1987); (14) Masuyama et al. (1989). The principal reason for apnea and periodic breathing during sleep in hypoxic environments is believed to be elevations in controller or feedback gain—often termed “control theory”—as evidenced by the steep increase in the CO2 response slope above and below eupnea and the greatly narrowed CO2 reserve (Ainslie et al. 2013; Dempsey 2005). Control theory can be used to better understand the central mechanisms responsible for periodic breathing (Cherniack and Longobardo 2006; Khoo et al. 1982). For example, control systems are marked by two key features: a “disturbance” (e.g., a change in alveolar ventilation) followed by a “corrective action,” which tends to suppress the disturbance. In the case of an increase in alveolar ventilation (caused by a sigh, for example), the corrective action would be a lowering of PaCO2, which would tend to reduce ventilation by its action on central and peripheral chemoreceptors and thus constitute negative feedback. Sustained oscillatory behavior will occur in such a system when two requirements are met. First, the magnitude of the corrective action must exceed that of the disturbance, this ratio being known as the loop gain. Second, the corrective action must be presented 180° out of phase with the disturbance, so that what would otherwise inhibit the change in ventilation now augments it. This sustained oscillatory behavior occurs when the loop gain exceeds unity at a phase difference of 180°. Control theory predicts that the higher the loop gain at a phase angle of 180°, the more likely periodic breathing is to occur, the more marked the pattern of periodic breathing, and the shorter the cycle length of the periodic breathing. The main factor increasing loop gain in acclimatized lowlanders at high altitude is the increased chemoreceptor gain, particularly the response to severe hypoxia (Chapter 9). However, as described in detail elsewhere (Dempsey et al. 2010), it should be noted that loop gain of the chemoreceptor control system is a product of two gains: 1) the controller gain (i.e., the ventilatory reflex gain, ΔV.E/ΔCO2); and 2) the plant gain (i.e., effectiveness of alveolar ventilation in imparting changes in blood gases, ΔCO2/ΔV.E). Although poorly understood, especially in humans, another gain—the mixing gain— may also influence breathing stability. The mixing gain is known as the effectiveness of brain blood flow (BBF) in imparting changes at the level of the central chemoreceptors (ΔCO2/ΔBBF). Another important factor that has been postulated to influence ventilatory stability and thus periodic breathing is the poststimulus short-term potentiation, or what was initially called the “ventilatory afterdischarge” (Eldridge 1977). Although these concepts have been described in detail with sleep apnea (Dempsey et al. 2010), an update is provided on the likely changes experienced at high altitude and the implications for periodic breathing. The transient cessation of the medullary respiratory pattern generator neurons requires an unmasking of a sensitized apneic threshold in NREM sleep, as induced by a transient ventilatory overshoot involving both mild to moderate hypocapnia plus augmented tidal volumes. In cats and rats, carotid body denervation studies demonstrate that the carotid bodies are required for sensing the low PaCO2 and causing ventilatory instability and cyclical apneas (Dempsey et al. 2010; Nakayama et al. 2003). However, hypocapnia induced at the level of both peripheral and central chemoreceptors is required to elicit apnea (Dempsey et al. 2012; Smith et al. 2007). Vagal blockade in sleeping animals also shows that inhibitory feedback from lung stretch accompanying transient increases in tidal volume contributes to the apnea following a ventilatory overshoot (Chow et al. 1994). These inhibitory effects on breathing are opposed by excitatory central short-term potentiation mechanisms which preserve ventilatory drive immediately following ventilatory overshoots while awake but apparently not during NREM sleep (Badr et al. 1992; Iber et al. 1986). Two additional mechanisms to enhance postapneic ventilatory overshoots include: (a) apneas are commonly prolonged until PaCO2 rises above its normal preapneic, eupneic level (Leevers et al. 1993); and (b) transient arousals at end apnea are common and will enhance the magnitude of the transient ventilatory overshoot response to chemoreceptor stimulation. These mechanisms underlie a sleep-induced apnea but the repeated, cyclical occurrence of transient ventilatory undershoots (apneas/hypopneas) and overshoots requires that subjects’ respiratory control system “loop gain” be elevated. The principle component to the high loop gain is a high chemosensitivity to CO2 both above and below the level of eupneic ventilation. This high gain means that both ventilatory undershoots in response to a transient hypocapnia and ventilatory overshoots in response to a combination of apneic-induced hypoxemic and hypercapnic chemoreceptor stimuli are excessive, thereby precipitating the continued breathing periodicity (Dempsey 2005; Khoo 2000). The concept of loop gain and its two main principle components—controller (CO2 chemosensitivity or ΔV.E/ΔPaCO2 slope) and plant gain (or ΔPaCO2/ΔV.E)—are illustrated in Figure 17.5 (Javaheri and Dempsey 2013). Figure 17.5Illustration of the relationship between alveolar ventilation and arterial PCO2 at a fixed CO2 production (e.g., 250 mL/min). Ascent to altitude increases the chemoreflex slope (solid blue line), but does not necessarily change the apnea threshold, and by doing so moves the equilibrium to an increased ventilation and lower PCO2, thereby decreasing plant gain. This chronic hyperventilation induced by the high altitude and subsequent reductions in plant gain indicates that a greater transient increase in V· A and therefore reduction in PaCO2 is required to reach the apneic threshold than it would be under conditions of normocapnia. A common misconception, particularly with reference to causes of periodic breathing in hypoxia, is that a reduced steady-state PaCO2 will precipitate periodic breathing, presumably because the eupneic PaCO2 is closer to their apneic threshold (Bradley and Phillipson 1992; Lorenzi-Filho et al. 1999). This is a misconception because the position of the apneic threshold relative to eupneic PaCO2 is labile; i.e., the CO2 reserve is determined by the chemosensitivity and slope of the CO2 response (Lahiri et al. 1983). Therefore, this reduction in plant gain acts to stabilize breathing. Should the apnea threshold also be decreased with acclimatization at altitude (dotted blue line), then ventilation increases and PCO2 decreases and plant gain is further decreased. For a given background PaCO2, alterations in the slope of the V· E−PaCO2 relationship below eupnea would alter the CO2 reserve (i.e., the amount of reduction in PaCO2 required to cause apnea). Changing the slope of the ventilatory response to CO2 above eupnea would alter susceptibility for transient ventilatory overshoots. Although it seems that at high altitude the chronic hyperventilation-induced hypocapnia may be “protective” against apnea and breathing instability via reductions in plant gain, the other chemoreceptor (e.g., controller gain) and nonchemoreceptor factors (e.g., increased pulmonary pressures, behavioral drives, awake-to-sleep transitions, locomotion feedback/forward stimuli, etc.) may contribute and potentially negate this response. (Modified from Ainslie et al. 2013.) Loop gain is increased in hypoxia or high altitude because chemoreceptor CO2 sensitivity is increased more than controller gain is reduced (see Figure 17.3). Thus the apneic threshold resides within 1–2 mmHg of the eupneic PaCO2 during NREM sleep, ensuring a significant ventilatory undershoot with even small levels of transient hypocapnia; further the ventilatory overshoot at apnea terminations are amplified because of the synergistic stimulatory effects on carotid chemoreceptors of hypoxemia plus hypercapnia (i.e., asphyxia) (Xie et al. 2001). Chemoreceptor sensory inputs drive both medullary rhythm generating neurons as well as arousal producing cortical neurons (Berssenbrugge et al. 1983; Khoo 2000). These combined oscillating powerful drives and inhibitors to breathing likely explain, respectively, the abrupt large ventilatory overshoots at apnea termination and abrupt ventilatory undershoots at end hyperpnea in hypoxic environments, resulting in the cluster type breathing pattern (see Figure 17.2). Interestingly, sex may influence the extent of periodic breathing (as indexed by the AHI) at high altitude; i.e., females have a reduced AHI compared with males at 5400 m (Lombardi et al. 2013). In another related study conducted at 4559 m, greater severity of periodic breathing during sleep displayed by men when compared to females at altitude has been reported to be attributed to their increased hypoxic chemosensitivity (Caravita et al. 2015). Nevertheless, acetazolamide counteracted the occurrence of periodic breathing at altitude in both sexes, modifying the apneic threshold and improving oxygenation (Caravita et al. 2015). The Tibetan Sherpa, a population well-adapted for superior performance at high altitude, tend to have little to no period breathing during sleep (Hackett et al. 1980; Lahiri et al. 1983). Figure 17.6 shows the relationship between the frequency of apnea during sleep at 5400 m and the hypoxic ventilatory response in these two groups. Because native highlanders often have a blunted hypoxic ventilatory response (Milledge and Lahiri 1967; Severinghaus et al. 1966), the loop gain of the control system is reduced and the factors promoting periodicity are weak. Lahiri et al. (1983) have argued that this represents an important feature of the true adaptation of native highlanders, such as Sherpas, to high altitude. In contrast, Andean natives, who have adapted considerably less than the Sherpa (see Chapter 4), especially those with chronic mountain sickness, still have marked periodic breathing (Bernardi et al. 2003; Guan et al. 2015; Julian et al. 2013; Moraga et al. 2014; Rexhaj et al. 2016; Sun et al. 1996). The observation is broadly consistent with the report that, in lowlanders, periodic breathing is not altered over 13 months at the Antarctic base Concordia, located at an equivalent altitude of 3800 m (Tellez et al. 2014). The implications and benefits of the attenuated period breathing during sleep in the Sherpa as an important phenotype of adaptation remain to be explored. Figure 17.6Relationship between frequency of sleep apnea and ventilatory response to hypoxia (awake). ⦁, acclimatized lowlanders; Δ, high altitude Sherpa; ▴, lower altitude Sherpa. One lowlander did not have periods of apnea, and the low altitude Sherpa showed periodic breathing. (From Lahiri and Barnard 1983.) Periodic breathing causes marked fluctuations in the arterial PaO2 and SaO2 with the severity of hypoxemia being a function of both the duration of apnea and the altitude at which the individual is sleeping. In the study of nocturnal periodic breathing carried out at an altitude of 6300 m during the 1981 AMREE expedition, the mean fluctuation in SaO2 between subjects was approximately 10% (West et al. 1986). It was shown that the SaO2
Introduction
Historical
Quality of sleep
Periodic breathing
Physiology of Sleep
Stages of sleep
Slow Wave Sleep
Rapid Eye Movement (REM) Sleep
Control of breathing during sleep
Alterations in normal sleep patterns
Characteristics of Sleep at High Altitude
Increased frequency of arousals
Changes of sleep state
Changes in control of breathing
Periodic Breathing
Characteristics
Persistence Over Time at High Altitude
Percentage of Time Occupied by Periodic Breathing
Periodic Breathing and Sleep Staging
Cycle Length
Mechanism(s) for periodic breathing
Sex Differences in Periodic Breathing
Periodic breathing in high altitude populations
Gas exchange
Stay updated, free articles. Join our Telegram channel
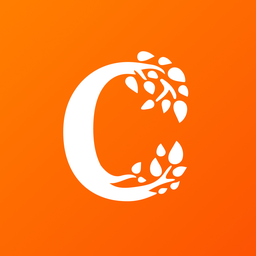
Full access? Get Clinical Tree
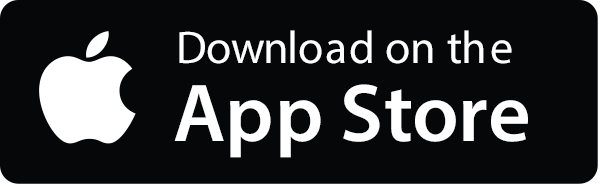
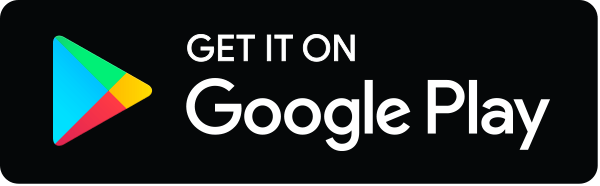