Background
The aim of this study was to investigate the systolic and diastolic properties of the right cardiac chambers (the right ventricle and right atrium) among different subsets of athletes to unveil potential variations in right ventricular and right atrial remodeling secondary to different training modes.
Methods
A cohort of Caucasian male top-level athletes ( n = 108; 80 endurance athletes [EAs], mean age, 31.2 ± 10.4 years; 28 strength-trained athletes [SAs], mean age, 27.4 ± 5.7 years) and untrained controls ( n = 26; mean age, 26.6 ± 5.6 years) ( P = .327) were prospectively enrolled. Conventional echocardiographic parameters, including transtricuspid inflow, Doppler tissue imaging, and two-dimensionally derived peak systolic longitudinal strain and strain rate indices of the right ventricle and right atrium, were calculated.
Results
EAs had greater internal right ventricular and right atrial dimensions compared with SAs and controls. There were no significant differences concerning strain between groups (−23.1 ± 3.7% in EAs vs −25.1 ± 3.2% in SAs vs −23.1 ± 3.5% in controls, P = .052), with SAs presenting higher global systolic strain rates (−1.42 ± 0.22 sec −1 in SAs vs −1.21 ± 0.21 sec −1 in EAs vs −1.2 ± 0.28 sec −1 in controls, P = .016), as well as greater right atrial strain rate systolic and diastolic components. Training volume (highly vs moderately trained athletes) did not significantly influence deformation parameters. No significant differences concerning diastolic transtricuspid inflow and Doppler tissue imaging indices were also noted among different athlete groups and controls.
Conclusions
Despite the existence of right geometric alterations in athletes participating in different sport disciplines, few meaningful differences in deformation and diastolic function exist.
Systematic training imposes a wide range of cardiovascular adaptations, both structural and functional, affecting both ventricles and atria. These physiologic changes occur to provide amplified cardiac output to satisfy increased metabolic demands during high-intensity workload. Although athletic training results in remodeling of all cardiac cavities, morphologic and functional adaptations of the right ventricle and right atrium have been given considerably less attention compared with those of the left ventricle and atrium, with a few recent studies focusing on geometric remodeling and systolic function of the right ventricle, based mainly on endurance athletes (EAs).
Post-training right ventricular (RV) conditioning is a complicated phenomenon potentially influenced by many different parameters. Sport discipline has been proved to play a major role in the configuration of athlete’s heart. Volume overload imposed on the heart chambers by endurance training leads to cavity dilatation and to an “eccentric” left ventricular (LV) hypertrophy pattern, whereas strength sports subsequently cause less eccentric hypertrophy. Taking into consideration the interdependence and close anatomic relationship between the two ventricles, along with the fact that a disproportionate load is placed on the right ventricle compared with the left ventricle during exercise, we would expect that apart from a different LV morphology, strength-trained athletes (SAs) potentially develop different RV and right atrial (RA) structural and functional adaptations compared with those observed in EAs, excessively studied till now. Additionally, the impact of training volume on RV morphology, deformation, and diastolic function and the existence of RA structure and functional variations among different subsets of athletes are other key features not clarified by existing studies.
Apart from its significance in understanding the physiology of exercise, the approach to RV and RA morphologic and functional adaptations to systematic training is important in preparticipation screening of athletes. Previous studies assessing RV function in EAs have found echocardiographic measures of myocardial function lower than those seen in nonathletes, concluding that reduced RV function might reflect myocardial pathology. Given the overlap between the athlete’s heart phenotype and that of arrhythmogenic RV cardiomyopathy (ARVC), especially among athletes presenting cardiac enlargement and frequent ventricular ectopy, it is essential to clarify deformation and diastolic function indices among healthy athletes of different subsets, attempting to find RV functional echocardiographic measures that may provide greater distinction between pathology and physiologic adaptation to exercise. Furthermore, assessment of RA deformation dynamics might be a useful tool in the study of right heart adaptations to exercise, as it may offer new indices for the discrimination of athlete’s heart from cardiomyopathic processes.
Taking into consideration these assumptions, we hypothesized that morphologic adaptations of right heart cavities in athletes are not accompanied by RV systolic and diastolic functional impairment. For this reason, we sought to test RV systolic and diastolic function indices among different subsets of athletes on the basis of sport discipline (EAs vs SAs), training volume (highly vs moderately trained athletes), and RV morphology (dilatation or not) and to compare the derived values with those of healthy untrained controls. Additionally, we aimed to provide a range of data for global RA strain (ε) and strain rate (SR) indices in the aforementioned groups of athletes.
Methods
Study Population
This was a prospective study designed and performed in Thessaloniki, in northern Greece, during the peak competitive seasons of 2011 and 2012. After contacting the National Federations of Sports as well as sports physicians and coaches of the top-level sports teams in northern Greece, asking to recruit athletes competing in national-level and international-level training in our region, 108 Caucasian male top-level athletes in different sport disciplines volunteered to participate in our study. All athletes had >3 years of training experience and exercised at least four times per week with ≥8 hours of weekly training workload. Our cohort consisted of 80 athletes involved in sports combining high dynamic and high static components, including endurance cycling, running, and triathlon, and 28 athletes performing tae kwon do, judo, and weightlifting, sports with high static and low dynamic demands. Athletes with ≥20 hours of training per week (the 75th percentile) were considered to be highly trained athletes (25% of EAs and 28.6% of SAs, P = .75), with moderately trained athletes having >8 and <20 hours of weekly training time. We adopted this cutoff because athletes in the upper quartile of training volume shared characteristics of highly trained athletes, as these have been described in previous reports. All enrolled subjects were free from known cardiovascular disease, had negative family histories of cardiomyopathies or premature sudden death, and were not systematically taking any form of prescribed medications or illicit drugs. None of the involved athletes presented with abnormal electrocardiographic features according to a previous European Society of Cardiology report. Even though some enrollees fulfilled dimensional criteria for ARVC, none had subjective evidence of the disease, such as saccular outpouching, wall motion abnormalities, or prominent moderator bands.
Additionally, 26 healthy nonathletic control subjects (training for <3 hours/week, with no previous training experience), served as a reference group, with the same exclusion criteria as used for athletes. All participants provided written informed consent at the time of enrollment, and the study was granted ethical approval by the research committee of the Aristotle University of Thessaloniki, Greece.
Echocardiographic Assessment
After complete personal and family medical histories, a careful physical examination, and 12-lead electrocardiography, the echocardiographic examination was performed, using a Vivid S5 scanner (GE Vingmed Ultrasound AS, Horten, Norway) with a broadband M3 S transducer. All echocardiographic examinations of participants were performed by the same experienced sonographer using the same standard protocol. EchoPAC dedicated software (GE Vingmed Ultrasound AS) permitted offline analysis of the studies.
Standard LV two-dimensional (2D) parameters were obtained from parasternal and modified apical acoustic windows, after all settings were optimized to achieve optimal endocardial delineation in accordance with American Society of Echocardiography guidelines, while for the right ventricle, the dimensions shown in Figure 1 were calculated. RA end-systolic area was obtained from an apical four-chamber orientation focusing on the right cavities. Body surface area (BSA) was estimated according to the formula of DuBois and DuBois. Following a previously described methodology and taking into account that the proposed cutoffs suggested by the American Society of Echocardiography are not corrected for gender, ethnicity, athletic involvement, and/or BSA, RV dilatation was defined as a BSA-corrected RV inflow diameter >1 standard deviation of the mean of the control population of the present study (>2.22 cm/m 2 ).

RV diastolic function was assessed both by evaluation of transtricuspid inflow parameters and by means of pulsed-wave Doppler tissue imaging (DTI), using a 5-mm sample volume placed in the RV lateral wall of the tricuspid annulus at end-expiration ( Figure 1 ). Three cardiac cycles were averaged for each parameter. Pulmonary artery systolic pressure was estimated according to RV assessment guidelines.
2D Myocardial Speckle-Tracking
For the acquisition of RV functional data, necessary for consequent 2D myocardial speckle-tracking analysis, the apical four-chamber orientation was used, and frame rates were as high as possible (not >90 frames/sec), with the focal point positioned at the midlevel of the RV cavity to minimize the impact of beam divergence. All images were optimized with gain, compression, and dynamic range to enhance myocardial definition with standardized depth, frequency, and insonation angle for all participants. For offline analysis, a region of interest was manually traced along the endocardial border from base to apex at the end of systole, and width was set to match the wall thickness. If the automated 2D analysis appraisal of acceptable tracking quality indicated inappropriate tracking, retracing was performed until all six RV myocardial segments (three septal and three RV free wall) were considered to be acceptable. The following parameters were measured: global longitudinal peak systolic ε as the average of all six RV myocardial segments, RV free wall longitudinal ε as the average of the three RV free wall segments, and basal RV free wall ε. Also, systolic SR (SRS’) along with early and late diastolic SRs were calculated as the average of the base, mid, and apical segments of the right ventricle ( Figure 2 ). Additionally, using a similar methodology tracing the endocardial border of the right atrium, the following parameters were estimated: peak atrial longitudinal ε (PALS) and peak atrial contraction ε were measured using a six-segment model for the right atrium. SR and its components (RA peak SRS’, RA early diastolic SR, and RA late diastolic SR) were also measured starting from QRS onset.

To minimize measurement bias, we randomly selected 70 of the total of 134 echocardiographic studies to reassess 2 days after initial analysis of each study. Intraobserver variation was estimated, because a single operator performed both data acquisition and measurement. During repeated measurements, the researcher was blinded to previous results. Intraobserver variability was expressed using the coefficient of variation.
Statistical Analysis
Analyses were performed using SPSS Statistics version 19.0 (SPSS, Inc., Chicago, IL).
Sample size was calculated using an α level of 0.05, power of 0.80, and (taking into consideration our regional athletic population sport disciplines’ distribution) a ratio of SAs to EAs of 1:3 to detect an absolute difference of 2 ± 3% in mean global RV ε between groups on the basis of literature data. Two groups enrolling ≥75 EAs and ≥25 SAs each were deemed adequate to meet the aforementioned criteria.
Data are expressed as mean ± SD for continuous variables and as counts and/or percentages for categorical variables. Comparison of variables was performed with one-way analysis of variance with post hoc Bonferroni analysis for groups of more than two. Chi-square or Fisher’s exact tests (for expected cell values < 5) were used to compare nominally scaled variables. Correlations between variables were assessed using Pearson’s r coefficient. In an attempt to minimize statistical bias due to unequal sample sizes or to violations of assumptions of the parametric tests used, bootstrapping analysis was performed, randomly resampling the study population 1,000 times. Comparisons’ P values reported are those adjusted from bootstrapping analysis.
Concerning intraobserver variability, the mean value of the two observations ( x ) performed for all the parameters for more than half of the echocardiographic studies and the absolute value of difference between observations ( e ) ± SD were determined. Reproducibility was assessed by the coefficient of variation: ( e / x )100%.
Results
Basic Demographic and Standard LV Morphologic Parameters
Age, anthropometric data, clinical characteristics, and performance measures of the study population are shown in Table 1 . No significant differences concerning age and body habitus parameters between athletes and controls were recorded. As expected, athletes presented, because of exercise-induced remodeling, greater internal dimensions (LV end-diastolic diameter and volume) and LV mass index compared with controls (LV end-diastolic diameter, 5.3 ± 0.35 cm in EAs vs 5 ± 0.38 cm in SAs vs 4.6 ± 0.5 cm in controls [ P = .005]; LV end-diastolic volume, 133.2 ± 21.2 mL in EAs vs 110 ± 17 mL in SAs vs 106.8 ± 16 mL in controls [ P = .001]; LV mass index, 108.9 ± 27.5 g/m 2 in EAs vs 91.8 ± 18.3 g/m 2 in SAs vs 82.7 ± 21 g/m 2 in controls [ P < .0005]). LV diastolic function remained well within “normal” limits, showing no significant differences with values obtained from nonathletes.
Variable | EAs ( n = 80) | SAs ( n = 28) | Controls ( n = 26) | P (ANOVA) |
---|---|---|---|---|
Age (y) | 31.2 ± 10.4 | 27.4 ± 5.7 | 26.6 ± 5.6 | .327 |
BSA (m 2 ) | 1.98 ± 0.15 | 1.95 ± 0.21 | 2.01 ± 0.16 | .333 |
BMI (kg/m 2 ) | 21.9 ± 2.5 | 21.2 ± 2.8 | 22.4 ± 2.4 | .353 |
HR (beats/min) | 50 ± 7 | 56 ± 6 | 65 ± 9 | <.001 |
SBP (mm Hg) | 120 ± 7 | 125.4 ± 8 | 120.6 ± 8 | .29 |
DBP (mm Hg) | 70.3 ± 8.5 | 78 ± 3 | 75 ± 9 | .25 |
Training experience (y) | 11.3 ± 8.3 | 10.3 ± 5.6 | NA | .488 |
Training volume (hours/week) | 14.6 ± 5.4 | 17.1 ± 7.2 | NA | .115 |
RV and RA Morphology and Function in EAs and SAs
Echocardiographic parameters concerning right cavity dimensions and functional data including transtricuspid inflow and DTI indices of EAs, SAs, and controls participating in our study are shown in Table 2 . Different training modes induced different morphologic RV patterns, leading to greater RV and RA dilatation among EAs, with 8.1% and 18.6% of them fulfilling major and minor dimensional criteria, respectively, for ARVC, but without other disease signs. There were no significant differences concerning ε between groups ( P = .085), with SAs presenting higher global SRS’, as well as greater RA SSR’ and RA late diastolic SR waves of RA SR and marginally nonsignificant higher PALS ( Table 3 ). Also, the comparison of global, RV free wall, and basal longitudinal ε between EAs and SAs is shown in Figure 3 .
Variable | EAs ( n = 80) | SAs ( n = 28) | Controls ( n = 26) | Bootstrapping analysis | P (ANOVA) | ||
---|---|---|---|---|---|---|---|
Controls vs EAs | Controls vs SAs | EAs vs SAs | |||||
Morphologic parameters | |||||||
RVOTd (cm) | 3.7 ± 0.5 | 3.4 ± 0.77 | 3.2 ± 0.66 | 0.001 | 0.927 | 0.087 | .001 |
RVITd (cm) | 4.1 ± 0.4 | 3.7 ± 0.4 | 3.8 ± 0.6 | 0.024 | 0.523 | <0.0005 | <.0005 |
RVDm (cm) | 3.1 ± 0.5 | 3.0 ± 0.5 | 2.6 ± 0.5 | <0.0005 | 0.017 | 0.887 | <.0005 |
RVL (cm) | 8.9 ± 0.6 | 8.5 ± 0.6 | 8.8 ± 0.7 | 1.00 | 0.517 | 0.113 | .114 |
RV EDA (cm2) | 26.6 ± 4 | 22 ± 3.5 | 19.2 ± 3.9 | <0.0005 | 0.037 | 0.001 | <.0005 |
RV ESA (cm 2 ) | 13.2 ± 2.7 | 10.5 ± 1.5 | 10.9 ± 2.4 | 0.074 | 1.00 | 0.006 | .016 |
RV FAC (%) | 50.4 ± 7 | 51.2 ± 9.8 | 42.7 ± 7.2 | 0.029 | 0.006 | 0.257 | .007 |
RA area (cm 2 ) | 17.5 ± 2.7 | 15 ± 3.6 | 14 ± 4.1 | <0.0005 | 0.844 | 0.005 | <.0005 |
Functional parameters | |||||||
Tricuspid E (cm/sec) | 66.9 ± 13.3 | 66.3 ± 13.6 | 68.7 ± 9.5 | 1.00 | 1.00 | 1.00 | .753 |
Tricuspid A (cm/sec) | 42 ± 11.2 | 38.9 ± 10.7 | 35 ± 5.7 | 0.007 | 0.488 | 0.448 | .008 |
E/A ratio | 1.64 ± 0.30 | 1.76 ± 0.42 | 1.98 ± 0.28 | <0.0005 | 0.031 | 0.195 | <.0005 |
DT (msec) | 141 ± 15,8 | 155 ± 14.4 | 145.3 ± 13.4 | 0.592 | 0.048 | <0.0005 | <.0005 |
RV FW S′ (cm/sec) | 15.3 ± 2.2 | 16.6 ± 2.1 | 14.8 ± 1.7 | 0.731 | 0.006 | 0.02 | .004 |
RV FW E′ (cm/sec) | 17.3 ± 2.7 | 18.1 ± 1.3 | 17.5 ± 2.9 | 1.00 | 1.00 | 0.364 | .299 |
RV FW A′ (cm/sec) | 10.7 ± 2.8 | 11 ± 2.2 | 10.2 ± 2.1 | 1.00 | 0.70 | 1.00 | .480 |
E/E′ ratio | 3.9 ± 1 | 3.6 ± 0.66 | 4 ± 0.85 | 1.00 | 0.497 | 0.419 | .192 |
PASP (mm Hg) | 21 ± 8.3 | 22.4 ± 8.6 | 18.3 ± 8.6 | 0.494 | 0.211 | 1.00 | .192 |
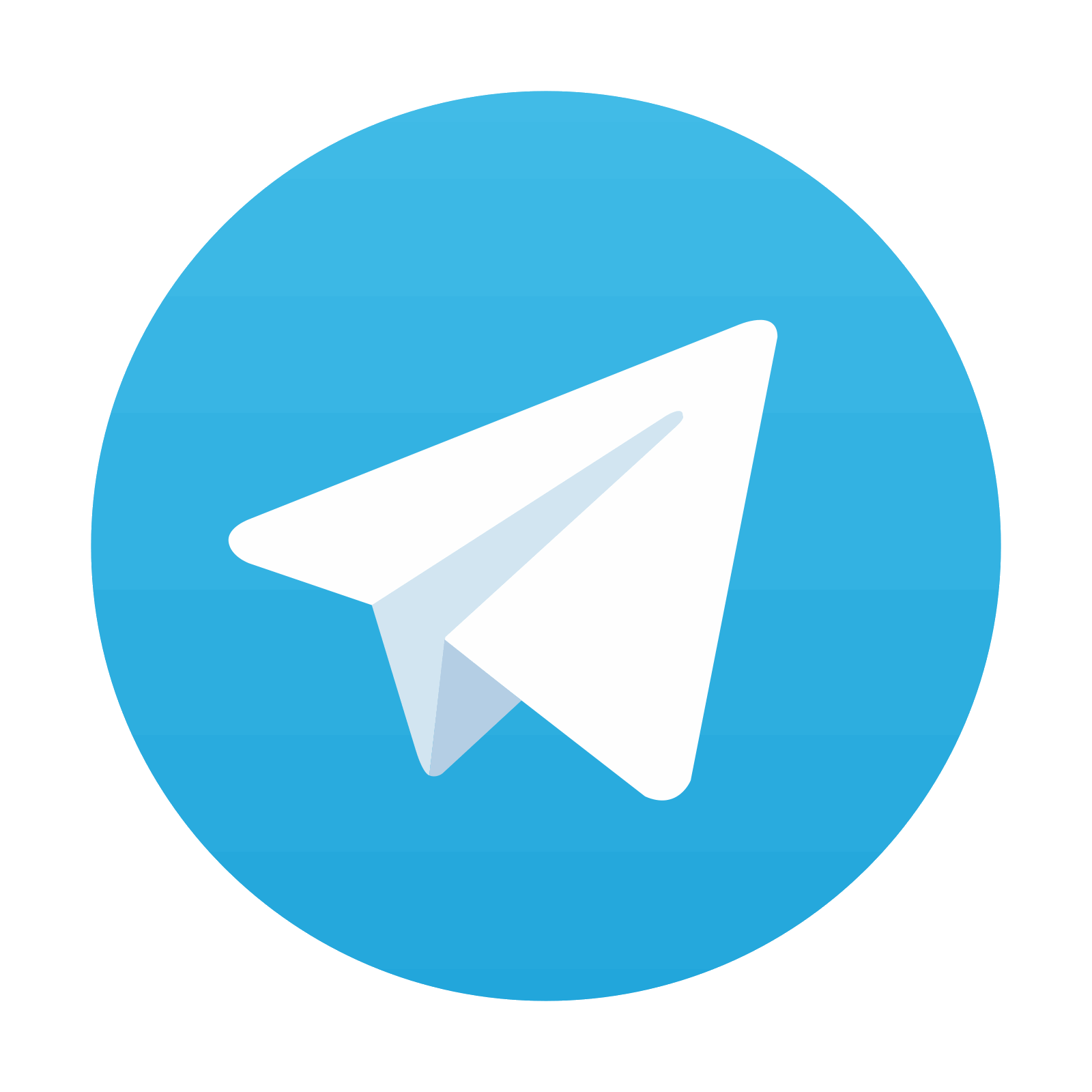
Stay updated, free articles. Join our Telegram channel
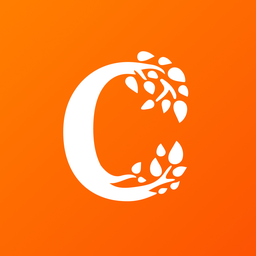
Full access? Get Clinical Tree
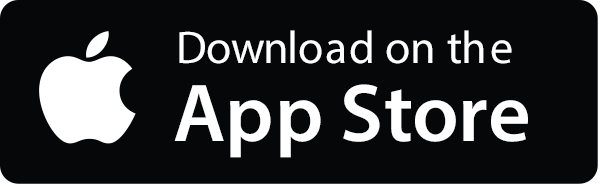
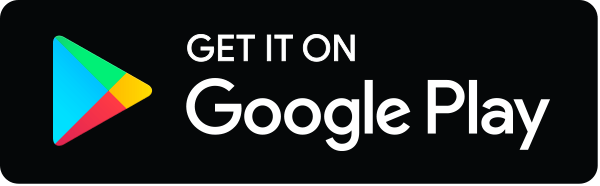
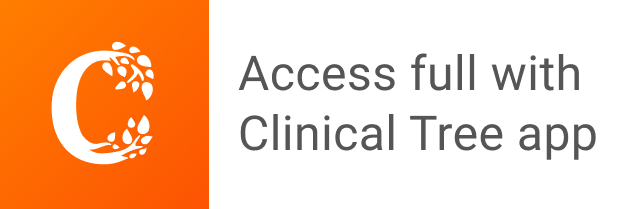