Abstract
The most common reasons for admission of newborn infants to the neonatal intensive care unit are respiratory disorders. Despite advances in neonatal intensive care, respiratory disorders remain an important cause of mortality and morbidity amongst newborns. As infants transition from an in utero to external environment, many changes in respiratory regulation and hemodynamic status must occur in order for the baby to adapt successfully. However, some babies may be unable to transition successfully and require resuscitation and further treatment. The changes in cardiopulmonary physiology can also be vulnerable to disruptions and may give rise to disorders such as periodic breathing or apnea of prematurity, in the case of premature infants. Common neonatal respiratory disorders include respiratory distress syndrome, transient tachypea of the newborn, meconium aspiration syndrome, pneumonia, and congenital airway abnormalities. In this chapter the mechanisms of respiratory control, indications and procedures for neonatal resuscitation, and the etiology, presentation, management, and outcome of common neonatal respiratory disorders will be described.
Keywords
congenital, neonatal, respiratory disorders, neonatal resuscitation, ventilatory control
Respiratory disorders are the most common reason for admission to a neonatal intensive care unit (NICU) and are a major source of neonatal mortality and morbidity. This chapter describes the etiology, presentation, management, and outcome of neonatal respiratory disorders, as well as the initiation of respiration at birth and resuscitation.
Initiation of Respiration at Birth
One of the most important and well-coordinated physiologic events in a person’s life is the transition from the fetal state to the newborn infant. Within the first minute of life, the newborn must clear the fetal lung fluid from the airways and establish gas exchange. Although most infants complete this transition without problems, inadequate initiation of respiration at birth can be catastrophic to both the respiratory and cardiovascular system.
The human fetus is breathing long before the infant is born, and fetal breathing is necessary for normal lung development. Fetal breathing is detected as early as 12–14 weeks gestational age (GA), and the frequency and duration correspond to the GA and sleep state of the fetus. Fetal breathing and swallowing occur during active sleep, with limited amounts during quiet sleep. During active sleep, the fetus has an irregular breathing pattern (20–30 bpm) characterized by long inspiratory and expiratory times with movement of fetal lung fluid into and out of the lung. Central chemoreceptors in the fetus respond to fetal hypoxia with decreased breathing (opposite of newborn physiology) and to hypercarbia by increased breathing and initiation of active sleep. Fetal breathing is probably controlled by prostaglandin secretion. Fetal sheep given prostaglandin E2 infusions stop breathing, and treatment with prostaglandin synthetase inhibitors, such as indomethacin, cause continuous fetal breathing. At birth, the rapid onset of regular breathing is likely due to a combination of removal of prostaglandin production from placenta, tactile and cold stimuli from the skin, activation of Hering-Breuer reflexes, and changes in arterial blood oxygenation levels (PaO 2 ). Most of term infants (85%) will begin spontaneous breathing by 10–30 seconds of life, with another 10% requiring only drying and stimulation to begin a regular breathing pattern. Most preterm infants <28 weeks and <1000 g (69%) have an audible cry and start breathing immediately after birth.
Fetal Lung Fluid
The airspaces of the fetal lung are filled with fluid created by the airway epithelial cells. Production and maintenance of the normal volume of fetal lung fluid is essential for normal lung growth. Fetal lung fluid is secreted by the airway epithelium as a filtrate of the interstitial fluid of the lung by the active transport of chloride. The fetal lung fluid is high in chloride but very low in protein and bicarbonate. Although the secretion rate has not been precisely measured in the human fetus, the fetal sheep during the middle of gestation has a secretion rate of about 4–5 mL/kg per hour. Fetal lung fluid flows intermittently up the trachea with fetal breathing because the pressure in the trachea exceeds the amniotic fluid pressure by approximately 1 cm H 2 O. Intermittent glottis closure in utero causes increased fetal lung fluid volume and pressure, and assists with lung development. Abnormal flow and pressure in the lungs during development lead to either lung hypoplasia (e.g., in oligohydramnios) or large dysplastic lungs (e.g., in chronic upper airway obstruction). The fetal lung is fully expanded near term with a fluid volume of about 40 mL/kg, which is approximately twice the functional residual capacity (FRC) of the air breathing newborn. As the pregnancy gets closer to term gestation, the rate of liquid formation and the volume of liquid within the fetal lung decreases.
Clearance of Lung Fluid From Airways at Birth
The fetal lung fluid is cleared through (1) active transport of sodium by epithelial sodium channels (ENaCs) during labor, (2) mechanical forces during birthing process, and (3) newborn breathing after birth. Although the fetal lung fluid is generated throughout gestation by active secretion of chloride through apical epithelial cells into the airways, in late gestation the number and activity of ENaCs increase and generate a Na + gradient in the lung’s interstitial space. Water follows the sodium out of the airspace through diffusion or through specific water channels (aquaporins). Aquaporins also increase throughout gestation and can be upregulated by antenatal steroids. Increases in fetal cortisol, thyroid hormones, and catecholamines near term gestation contribute to the reversal of fluid movement in the lungs. In preterm fetal sheep, infusion of cortisol and T 3 will activate the sodium pump, which normally occurs at term. Exposure to postnatal oxygen tension also increases sodium transport across the pulmonary epithelium. Blockage of or genetic mutations of the alpha-subunit of these amiloride-sensitive ENaCs leads to respiratory failure and death due to inability to clear fetal lung fluid. The critical role of ENaCs at birth has been questioned by the lack of respiratory distress at birth in mice missing the other two subunits of the ENaC. Cyclic nucleotide-gated channels on the type I pneumocytes also assist in Na transport out of cells. Overall, activation of the ENaC assists in fluid clearance from the lungs at birth and throughout life.
Although there is some evidence for a small amount of lung fluid to be cleared during the progression through the vaginal vault, most lung fluid excreted through the nose and mouth, when the infants head is exposed, is due to fetal repositioning and spinal flexion caused by uterine contractions. It is difficult to quantify the percent of lung fluid excreted by these physical mechanisms, but the combined relative contribution to fluid clearance is likely as high as 33% of lung fluid. Elective cesarean sections (C-sections), without labor or rupture of membranes, will not have this physical clearance of lung fluid or activation of ENaCs prior to initiation of breathing.
Even with the reduction of fetal lung fluid volume during late gestation and labor and the expulsion of fluid by spinal flexion, there is greater than 15 mL/kg of fluid in the airways at the time of birth. This fluid is cleared from the airways and driven into the interstitial space surrounding the airway within the first few seconds of life by a few large, negative pressure breaths. An FRC is formed following the first breath in normal term infants. Using phase-contrast x-rays, Hooper and coworkers demonstrate that fluid leaves airways during inspiration with some reflooding during expiration ( ). Positive end-expiratory pressure (PEEP) can decrease the backflow of interstitial fluid into airways, improve the uniformity of lung recruitment, and decrease the injury from mechanical ventilation. The components of fetal lung fluid then are cleared directly into the vasculature or via lymphatics from the lung interstitium over multiple hours.
Respiratory Depression at Birth
Although most infants initiate respirations at birth, failure can occur because of fetal hypoxia (birth asphyxia), maternal medications leading to suppression of breathing, congenital malformations, or failure of the central nervous system chemoreceptors. The combination of immature lungs and reduced respiratory drive makes preterm infants more likely to need assistance with breathing at birth. Primary apnea occurs in newborns after birth and often responds to tactile stimulation. In utero the fetus responds to hypoxia with decreased fetal respiration thereby decreasing metabolic requirements; thus a severely hypoxic infants will not breath at birth. Many of these infants will respond to stimulation and continuous positive airway pressure (CPAP). Room air (21%) has been shown to be preferable to 100% oxygen and is recommended for the initial resuscitation of the term infant. If the infant in primary apnea is not assisted and hypoxia continues, the infant progresses into secondary apnea, where the heart rate (HR) decreases and responds only to positive-pressure ventilation (PPV). Continued deterioration of blood pressure will follow and metabolic acidosis will develop if respiration is not initiated. PPV through either a face mask or endotracheal (ET) tube should restore cardiopulmonary function in the majority of infants, as less than 1% of infants should need epinephrine in the delivery room. Pulse oximetry is now recommended for any infant suspected of needing resuscitation. Apgar scores, named after Virginia Apgar, are assigned to the infant at 1 minute, 5 minute, and 10 minutes for HR, breathing, color, reflex, and tone. Although a useful guide for how a newborn resuscitation is progressing, there is a high degree of variability in the Apgar scores assigned to infants and color has recently been removed from the algorithm for neonatal resuscitation. Persistently low Apgar scores, especially <5 at 10 minutes, have been associated with higher infant mortality, cognitive delays, increased risk of cerebral palsy and increased educational needs throughout childhood. Infants with severe birth asphyxia, metabolic acidosis, and neurologic signs of encephalopathy should receive therapeutic hypothermia. Birth asphyxia can affect all the organ systems and management will be individualized based on laboratory findings.
Delivery Room Resuscitation
Approximately 10% of infants require some form of assistance at birth; approximately 2% require intubation and PPV, and less than 0.5% require chest compressions and epinephrine. The percent of infants requiring resuscitation increases with decreasing GA. Every 5 years, the International Liaison Committee on Resuscitation (ILCOR) reviews all studies on newborn resuscitation and provides treatment guidelines for infants who fail to breathe adequately at birth; the following comments reflect their most recent recommendations from 2015 ( Fig. 19.1 ). The new guidelines stress the importance of the first “golden” minute of life, when breathing should have spontaneously begun or respirations are being assisted. All newborn infants, including nonvigorous ones exposed to meconium, should receive stimulation and drying at birth. Routine oropharyngeal suction should not be done unless the oral pharynx appears occluded. If the infant is not breathing, is gasping, or HR is less than 100 at 30 seconds of life, the infant should receive PPV with PEEP via a T-piece or resuscitation bag. Resuscitation with room air is recommended for term infants, whereas most preterm infants can be resuscitated with 0.21–0.30 fraction of inspired oxygen (FiO 2 ). Pulse oximetry should be applied and the oxygen titrated to maintain infants within the normal ranges during the first minutes of life. It should be noted that the fetal PaO 2 is low and normal transitional oxygen saturations are not greater than 85% for almost 5 minutes. If after 30 seconds of PPV ventilation, with an initial pressure of 20–25 cm H 2 O, the HR is not greater than 100, the resuscitator should progress through a series of ventilation corrective steps (i.e., MR.SOPA— M ask adjustments/ R eposition airway/ S uction and O pen mouth and nose/increase P ressure/consider A lternative airway) and consider intubation of the infant. Intubation should be confirmed by chest rise, condensate in ET tube, improved HR, and carbon dioxide detector (PediCap). If HR remains less than 60 bpm after intubation, chest compressions should be started using a two-handed encircling chest approach. The sternum is compressed approximately a third the diameter of the chest (approximately 2 cm) at a ratio of 3 : 1 for 120 events (90 compressions and 30 breaths) in a minute. Pulse oximetry and a three-lead electrocardiography (ECG or EKG) can be used to help assess the HR. If adequate ventilation and compressions do not return HR greater than 60, then the infant should receive intravenous (IV) epinephrine (0.01–0.03 mg/kg) through an umbilical venous catheter (UVC) or IV catheter. ET epinephrine (0.05–0.1 mg/kg) can be tried until IV access is obtained but has a decreased response rate compared with IV epinephrine. The use of sodium bicarbonate and naloxone are no longer recommended during the newborn resuscitation. Normal saline or group O Rh-negative blood (10 mL/kg body weight) should be given over 5–10 minutes if the infant is hypotensive due to blood loss. If there is no HR and Apgar score is 0 after 10 minutes of adequate resuscitation, it is appropriate to discontinue further resuscitation due to the poor neurologic outcome. Because most infants will respond to appropriate ventilation, resuscitators should consider other underlying conditions that might impede ventilation, such as pneumothorax, hypoplastic lungs from oligohydramnios, congenital diaphragmatic hernia (CDH), or other congenital anomalies.

Resuscitation of the Preterm Infant
The GA of the preterm infant will determine many of the steps taken during resuscitation. The care of late preterm infants (34–37 weeks) often follows the resuscitation guidelines for term infants, but they should be monitored closely after birth due to increased newborn morbidities (jaundice, temperature control, transient tachypnea of the newborn [TTN], feeding). Infants less than 32 weeks have immaturity of most organ systems, with the lungs and brain being the most vulnerable to injury and most likely to affect resuscitation. Antenatal steroids prior to preterm delivery stimulate maturation of many of the organs. Fortunately, more than 90% of preterm infant less than 34 weeks GA will receive some portion of a steroid course prior to delivery and this improves response to resuscitation. The extremely immature brain may have decreased respiratory drive requiring PPV ventilation. Thermoregulation is extremely important for the very preterm infant, due to brain immaturity and lack of brown fat sources, and they ought to be placed in a plastic bag or thermal wrap immediately after delivery.
Lung immaturity has the largest influence on the resuscitation of the preterm infant, and many preterm infants require assistance in the transition from fetal life. Lung development, maturation, and the surfactant system are covered extensively in other chapters in this book. Although the lack of labor may lead to increased fetal lung fluid at birth (see earlier), antenatal steroids or the maternal conditions that led to preterm birth may have primed the preterm lung for gas exchange. Although increased surfactant levels are probably not present until closer to 48 hours after antenatal steroids, preterm sheep lungs have decreased thickness of the lung parenchyma by 15 hours (likely due to decreased interstitial fluid) and decreased injury from mechanical ventilation by 24 hours after steroids. Surfactant-deficient preterm babies may lack the muscle strength to generate sufficient negative pressure to overcome their compliant chest wall and high airway surface tension. The amount of surfactant stored in type II cells is low in preterm infants not exposed to antenatal steroids, so less is available for secretion in response to birth. Without the assistance of surfactant in reducing surface tension, it is difficult for very small infants to develop an FRC. Since the approval of exogenous surfactant replacement therapy in the early 1990s, there has been a trend towards intubation and treatment of the smallest infants with surfactant. It should be noted that all the surfactant studies were performed prior to the American College of Obstetricians and Gynecologists (ACOG) recommendation for antenatal corticosteroids in 1994, so it is unclear what the results would be in the current era. Multiple studies comparing CPAP and surfactant treatment have been conducted in the past 10 years, and each has shown a trend towards improved respiratory outcomes in the infants receiving CPAP. Meta-analysis of all the trials demonstrated approximately a 10% reduction in bronchopulmonary dysplasia (BPD) with early CPAP. Recently it has been recommended to attempt to support preterm infants with CPAP if they are breathing in the delivery room. Remarkably, almost 50% of infants born less than 750 g will be successful on CPAP alone. This is possible because an endogenous surfactant pool size of only approximately 5 mg/kg (5% of term levels) is necessary for preterm lambs to respond to CPAP and lambs with approximately 10% the endogenous pool size have reduced injury from mechanical ventilation. If given the opportunity, the majority of very preterm infants will successfully initiate breathing without extensive resuscitation. Extremely preterm infants that require extensive resuscitation in the delivery room have significant morbidities and increased mortality.
Although there are clear benefits to resuscitation of term infants with room air, the consensus is less clear on the starting oxygen levels for preterm infants. The 2015 guidelines recommend starting preterm infants at a FiO 2 between 0.21 and 0.30 and then titrating oxygen based on pulse oximetry. Preterm infants resuscitated with room air often require an increase in FiO 2 to maintain saturations. Studies comparing 30%–90% oxygen for preterm infants demonstrated that most infants in the lower oxygen group had an increased oxygen requirement to 40%, whereas the higher group had decreases in oxygen requirements. Eventual oxygen requirements ended up at approximately 30% oxygen in both groups.
To overcome the resistance created by the air-fluid interfaces in small airways of preterm infants, physicians have used prolonged inspiratory times, commonly called sustained inflation (SI), to recruit FRC. In preterm lambs, SI augmented the cardiorespiratory transition at birth and improved the HR response to resuscitation after asphyxiation. In a few small human studies, SI at birth decreased the need for mechanical ventilation at 72 hours and may lead to a decrease in BPD. In preterm infants, SI generates an average FRC volume recruitment of only 8 mL/kg and lung volume recruitment occurs only in spontaneously breathing infants. Adduction of the glottis, a normal fetal occurrence, blocks airflow in the nonbreathing infants. Multiple clinical trials are currently underway to determine if clearing airway fluid with an SI to achieve FRC will benefit preterm infants. ILCOR removed the recommendation for three brief SIs (added in 2010) from the 2015 guidelines until results are known.
Ventilatory Control
Complex networks of respiratory neurons within the medulla and pons generate and regulate the involuntary component of respiratory rhythm. Specifically, studies suggest that this spontaneous inspiratory rhythm originates from pacemaker neurons within the pre-Bötzinger complex, one of the networks of neurons that make up the ventral respiratory group in the ventral medulla. The phasic transition from inspiration to expiration is modulated by signals from the stretch receptors in the lungs and airways via the vagus nerves and neurons in the pneumotaxic and apneustic centers of the pons. The rhythm is transmitted to the cervical and thoracic spinal motor neurons that innervate the diaphragm and intercostal muscles. These descending pathways activate the inspiratory muscles while inhibiting the expiratory muscles. Breathing pattern is further influenced by afferents from the forebrain, hypothalamus, central and peripheral chemoreceptors, muscles, joints, and pain receptors. Most areas contain multiple neuromodulators that are partly released from the same neurons, implying that neuromodulation is integrated at many levels.
Several neurotransmitters play important roles in respiratory regulation. Glutamate is an excitatory neurotransmitter that activates receptors involved in generating and transmitting respiratory rhythms to spinal and cranial respiratory neurons. Serotonergic neurons in the medulla oblongata are part of a critical system that modulates autonomic and respiratory effector neurons. The most consistent effect of serotonin (5HT) is to restore a normal breathing pattern after hypoxic or ischemic insult. Opioids (endorphins and exogenous drugs) suppress respiration by peripheral and central actions; the latter are due to suppression of recurrent excitation by glutaminergic input within the primary respiratory network. Both γ-aminobutyric acid (GABA) and glycine are essential for generating respiratory rhythm; they are released by late and postinspiratory neurons and inhibit inspiratory neurons, thus facilitating the transition from inspiration to expiration. Deficiency of glycinergic inhibition in knockout mice results in a slower frequency of breathing.
Central and peripheral chemoreceptors are core components of the chemical regulation of breathing patterns. These chemoreceptors modify respiratory activity in response to changes in oxygen, carbon dioxide, and acid-base balance. The central chemoreceptors are situated near the ventral surface of the medulla, whereas the peripheral chemoreceptors are situated at the bifurcation of the common carotid arteries (carotid bodies) and above and below the aortic arch (aortic bodies). In the fetus the arterial chemoreceptors are active but have reduced sensitivity. The peripheral chemoreceptors are not essential for the initiation of respiration and are virtually silenced when the arterial oxygen level rises at birth. Resetting of the carotid chemoreceptors to hypoxia occurs within 24–48 hours of birth ; this may result from changes in dopamine levels. The fetus responds to hypoxia with a suppression of ventilation. The lateral part of the lower pons mediates the hypoxic suppression of ventilation. In response to hypoxia, there is a redistribution of the circulation to favor the heart, brain, and adrenals. This minimizes oxygen consumption and conserves oxygen supplies for vital organs. The newborn has a biphasic response to hypoxia, a transient increase in minute ventilation followed by a decrease to or below baseline levels. The initial increase in ventilation may be due to activation of peripheral chemoreceptors and reconfiguration within the pre-Bötzinger complex leading to a change from eupneic rhythm to a gasping pattern. The subsequent reduction in ventilation may result from a fall in carbon dioxide tension following the initial hyperventilation or the suppressant effect of hypoxia, which occurs in the fetal state and persists into the neonatal period. Very immature infants respond to hypoxia by becoming apneic, which is like the fetal response. The biphasic response to hypoxia disappears by 12–14 days, and the adult pattern is subsequently observed (i.e., stimulation of breathing without depression). However, an animal model suggests that the acute hypoxic ventilatory response of an individual could be attenuated by exposure to sustained hypoxia followed by chronic intermittent hypoxia during the neonatal period. In contrast, exposure to hyperoxia causes a temporary suppression of breathing due to withdrawal of peripheral chemoreceptor drive. After a few minutes of hyperoxia, ventilation increases to greater than control levels probably because of hyperoxic cerebral vasoconstriction resulting in increased brain tissue carbon dioxide. Immaturity and prolonged exposure to supplementary oxygen reduce the response to hyperoxia. The fetus and newborn respond to increased carbon dioxide levels with an increase in breathing activity. The slope of ventilatory response to carbon dioxide is less in term infants during active than quiet sleep, but it increases with postnatal growth. Other chemoreceptors include subepithelial chemoreceptors in the trachea, bronchi, and bronchioles that respond by changing the frequency and depth of respiration after exposure to toxic gases such as nitrogen dioxide and sulfur dioxide. This response is decreased during active sleep and in the premature infant.
Respiratory activity is also regulated by nonchemical means, such as stimulation by the many respiratory reflexes. Hering and Breuer described three respiratory reflexes: inflation, expiratory, and deflation. The Hering-Breuer inflation reflex is stimulated by lung inflation and results in cessation of respiratory activity. In the newborn the reflex produces a pattern of rapid, shallow tidal breathing and operates within the tidal volume range. In older subjects the reflex prevents excessive tidal volumes and can only be stimulated if the inflating volume is increased greater than a critical threshold. The Hering-Breuer expiratory reflex is stimulated if inflation is prolonged; the active expiration seen in infants ventilated at slow rates and long inflation times, and which may result in pneumothoraces, may be a manifestation of this reflex. In animal models the Hering-Breuer deflation reflex is evidenced by a prolonged inspiration generated in response to deflating the lung rapidly or following an unusually vigorous expiratory effort that takes the lung below its end-expiratory level. In the newborn, this reflex may have a role in maintaining the FRC. Head’s paradoxical reflex, also called the inspiratory augmenting reflex or provoked augmented inspiration, is the underlying mechanism of the first breath and sighing. A rapid inflation stimulates a stronger diaphragmatic contraction. The reflex improves compliance and reopens partially collapsed airways ; it has an important role in promoting lung expansion during resuscitation. Rapid chest wall distortion via the intercostal phrenic inhibitory reflex results in a shortening of inspiratory efforts. This reflex response is inhibited by an increase in FRC or applying CPAP. The mechanism may improve chest wall stability. The airway is protected from bronchospasm induced by cold exposure by upper airway reflexes that can increase upper airway resistance and decrease inspiratory air flow. The laryngeal afferents defend the lower airway from inadvertent inhalation of foreign bodies, with maturation of the laryngeal reflex being characterized by an increase in coughing and a decrease in swallowing and apnea. For a discussion of ventilatory control in sleep-disordered breathing, please refer to Chapter 81 .
Apnea
Apnea is defined as cessation of breathing for at least 20 seconds or at least 10 seconds if associated with oxygen desaturation or bradycardia. Apneas are classified as central, obstructive, or mixed. Most apneas are central in nature (46%–69%), with the rest being either purely obstructive (6%–12%) or a combination of central and obstructive (i.e., mixed) (20%–44%). Central apnea is characterized by cessation of inspiratory efforts in the absence of upper airway obstruction. In contrast, in obstructive apnea, despite the presence of central signals for breathing and the corresponding respiratory muscle activities, there is an absence of airflow through the airways due to upper airway obstruction. Bradycardia may occur within a few seconds of onset of apnea with accompanying disturbances in blood pressure and cerebral blood flow velocity. In infants without adequate cerebrovascular autoregulation, cerebral perfusion may decrease to very low levels during prolonged apnea and potentially exacerbate hypoxic-ischemic brain injury. The incidence of apnea of prematurity is inversely associated with GA and usually resolves by approximately 37–40 weeks postconceptional age.
Frequent episodes of apnea that respond to gentle stimulation may be considered normal for preterm infants. However, additional investigations and treatment are required if the apneas become frequent and/or associated with prolonged desaturation, because there are several potentially treatable associated factors that may be present (e.g., infection, intracranial abnormality or hemorrhage, anemia, metabolic disorders [e.g., hypoglycemia], temperature instability, and gastroesophageal reflux [GER] [see later in the chapter]). In addition, several pharmacologic agents (e.g., benzodiazepines and opioid analgesics) can also cause apnea. After excluding other treatable conditions, neonatal apnea may be treated with methylxanthines (e.g., theophylline) and caffeine, which have all been shown to reduce apnea in preterm infants, possibly by increasing the chemoreceptor sensitivity to carbon dioxide. Caffeine is the preferred agent in view of its relatively long half-life, which allows once-daily dosing, and its higher therapeutic index compared with theophylline. Side effects of theophylline include hyperactivity, tachycardia, cardiac dysrhythmias, feeding intolerance, and seizures. Caffeine treatment in infants with, or at risk for, apnea of prematurity has also been shown to reduce BPD and improve survival without neurodevelopmental delay. In addition, prophylactic caffeine reduces apnea/bradycardia and episodes of oxygen desaturation in preterm infants following postoperative anesthesia. Doxapram, a respiratory stimulant that is thought to act via the peripheral chemoreceptors, has been used to treat apnea in newborns but has been associated with many side effects (e.g., elevated blood pressure, abdominal distension, irritability, increased gastric residuals, and emesis). Furthermore, the long-term effects of this drug have not been adequately investigated. Relatively novel treatments include olfactory stimulation, inhalation of low-dose carbon dioxide, and limb proprioception stimulation. However, although some of these treatments show initial promise, whether they can be replicated in a wide variety of clinical situations and show any long-term advantages remain to be seen. Noninvasive ventilatory support (e.g., CPAP, noninvasive positive-pressure ventilation [NIPPV], and high-/low-flow nasal cannulas) may be considered for infants with frequent or prolonged apnea despite treatment with caffeine. The frequency of apnea has been shown to be decreased by increasing the FRC, stabilizing oxygenation, or by splinting the upper airway. Positive pressure support of the airway may be particularly effective in infants whose episodes are precipitated or prolonged by relative upper airway obstruction as both the pharynx and laryngeal aperture could be distended to reduce the risk of mixed and obstructive apneas. However, a neural component to the effects of positive pressure/flow has been suggested as even delivery of low-flow air has been shown to be effective, suggesting the possibility of the stabilization of the mechanoreceptors in the upper airway by the airflow. Infants with severe and refractory apnea unresponsive to the relatively noninvasive modalities of treatment would need to be intubated and supported by mechanical ventilation.
Periodic Breathing
Periodic breathing is a common breathing pattern that, unlike apnea of prematurity, can occur in infants of all GA. In contrast to apnea of prematurity, periodic breathing does not usually occur within the first 48 hours of life and can last for 6 months or longer. The literature suggests that among healthy term babies, 78% exhibit periodic breathing within the first 2 weeks of life, decreasing to 29% by 1 year of age. The definition of periodic breathing can be arbitrarily defined as three episodes of apnea of more than 3-second duration each that are interrupted by periods of breathing that are 20 seconds or less. The etiology of periodic breathing is thought to be increased sensitivity of chemoreceptors involved in ventilatory control, leading to overregulation of the breathing pattern in response to small changes in PaO 2 or PaCO 2 levels. This overcompensation leads to periodic overshooting of the target to be compensated for and the oscillation between apnea and normal breathing that is observed in this condition. Periodic breathing has previously been thought to be a benign condition. However, with improvements in computing power, detailed longitudinal analyses of neonatal breathing patterns have been made, and showed that preterm infants often exhibit increased percentage of time spent in periodic breathing before a diagnosis of necrotizing enterocolitis (NEC) or septicemia is made. Furthermore, in another longitudinal study of preterm infants, it has been found that periodic breathing can be associated with significant desaturations and reduction in cerebral oxygenation, especially during sleep. Although the use of CPAP or caffeine may be associated with less periodic breathing, because the long-term clinical implications of these events are unknown, further follow-up studies are warranted before evidence-based diagnostic or treatment recommendations can be made.
Respiratory Distress Syndrome
Respiratory distress syndrome (RDS), also referred to as hyaline membrane disease, is a respiratory condition that occurs in many preterm infants at birth and is defined by clinical and radiographic descriptions. Infants with RDS develop oxygen requirements within 6 hours of life, typically require respiratory support at 24 hours of life, and a have a chest x-ray with findings consistent with RDS. The severity of RDS has decreased with the introduction of surfactant and antenatal steroids, so the classic hyaline membranes, seen at autopsy, rarely occur in our current premature infants. Because many infants are ventilated in the delivery room and given surfactant, the radiologic evidence of air bronchograms may not be present. And some infants will be maintained on mechanical ventilation due to immature respiratory drive, yet have mature lungs with plenty of surfactant. Because it is difficult to define RDS in the era of surfactant therapy and antenatal steroids, there will be variations in the number of preterm infants with RDS across studies. Some may argue the alternative name for RDS may have changed from “hyaline membrane disease” to “respiratory instability of prematurity,” a phrase created by Bancalari and Jobs to describe the extremely preterm infants born in recent years.
Epidemiology
It is difficult to determine the exact incidence of RDS because multiple definitions exist. The incidence of RDS decreases as the GA increases. Prior to the introduction of antenatal steroids (1983–86), the incidence of RDS was: >95% at 25 weeks GA (average weight 800 g); 75% at 28 weeks (average weight 1000 g); 50% at 30 weeks GA (average weight 1400 g); and still approximately 20% at 34 weeks GA. Antenatal steroids have been shown to reduce RDS by an average of 40% in randomized trials. In the 1990s, when the more routine use of antenatal steroids was beginning (ACOG recommended steroid use in 1994), the incidence of RDS was approximately 70% between 500 and 1500 g. Of note, in the Vermont Oxford network, the antenatal steroid rate increased from 23% in 1991 to 72% in 1999, but there was no difference in the rate of RDS. Between 1997 and 2003, in the era of moderately high antenatal steroid use, approximately 63% of infants between 500 and 1000 g have RDS. Although antenatal corticosteroids have traditionally been given to mothers at risk of preterm birth prior to 34 weeks GA, a randomized trial demonstrated a benefit of corticosteroids in late preterm infants. The rates of RDS vary between races and sex. Female infants have a lower incidence of RDS than do male infants at all GAs, which is consistent across most mammalian species. Late preterm males have an odds ratio (OR) of 1.68 of having RDS compared with their female counterparts. Race plays an important role in the development of RDS, with African-American infants having a lower rate than do white infants. In one study, only 40% of African infants less than 32 weeks GA developed RDS compared with 75% of the white infants. Genetic variability as a risk factor for RDS has been confirmed in twin studies, in which monozygotic twins were more likely than dizygotic twins to both have RDS. The largest influences on development of RDS are GA, gender, race, and antenatal steroids. Genetic polymorphisms of surfactant proteins have been found to be strongly associated with risk of RDS in preterm infants (see Chapter 57 ).
Pathophysiology
RDS is due to structural immaturity of the preterm lung and a deficiency in surfactant pool size. Increased lung fluid, inadequate respiratory efforts, and underdevelopment of the chest wall and respiratory muscles also contribute to the infant’s inability to maintain expansion of the distal airspaces. Surfactant production and maturation are covered in detail in Chapter 5 , so only a summary will follow. Surfactant is produced in alveolar type II cells, which are cuboidal cells covering approximately 2% of the alveolar surface that become more prominent between 22 and 24 weeks GA. Surfactant is a complex mixture of phospholipids, neutral lipids, and proteins (surfactant proteins A, B, C, and D). Lipids make up more than 90% of surfactant, with 80% being phospholipids (phosphatidylcholine [PC] and phosphatidylglycerol [PG] make up largest portion) and 10% neutral lipids (cholesterol, triacylglycerol, and free fatty acids). Sphingomyelin, which is used in the lecithin to sphingomyelin (L:S) ratio, represents less than 2% of surfactant lipid. Surfactant is synthesized in the endoplasmic reticulum, collected in the lamellar bodies then released into airspace as active tubular myelin. The tubular myelin mixes with a combination of saturated and unsaturated phospholipids to make a surface film that decreases surface tension. The surface film is recycled over time by the type II cells or degraded by the macrophages. More than 90% of the PC on the alveolar surface is reprocessed, the turnover time being approximately 10 hours. The phospholipid monolayer structure allows the surfactant to change shape throughout respiration, with the most distended form allowing decreased surface tension and the most compressed form in exhalation providing stability to the alveoli. In RDS the immature lung has a lower level of PG and PC, the spreading agents for surfactant in the lung, thus the monolayer formed is unstable and does not decrease surface tension or maintain alveolar patency as well. The immature surfactant system in preterm infants with RDS leads to difficulty in generating an FRC and increased work of breathing. The addition of PEEP or treatment with exogenous surfactant allows for formation of an FRC and decreased injury from ventilation. In RDS the resultant alveolar hypoventilation results in ventilation perfusion imbalance and hypoxia. The increased alveolar surface tension from the surfactant deficiency promotes the flow of protein-rich fluid from the intravascular space to the alveolar spaces. The leak of protein is increased by mechanical ventilation and from continued hypoxic damage to the alveolar-capillary membrane. These plasma proteins further inhibit surfactant function and make up a major component of the hyaline membrane found in infants who do not survive RDS.
The four surfactant-associated proteins, SP-A, SP-B, SP-C, and SP-D, compose approximately 10% of surfactant and play a role in the structure of tubular myelin (SP-A), the stabilization of the phospholipid monolayer (SP-B, SP-C), and in host-defense (SP-A, SP-D). ABCA3 is a protein that assists in transport of lipids with type II cells and is required for proper lamellar body formation. Genetic mutations in the surfactant proteins or ABCA3 lead to respiratory disease in the newborn that ranges from mild (SP-A) to lethal (SP-B). Some of the clinical manifestations of these conditions do not appear until childhood or adulthood. The role of gene mutations in human lung diseases and surfactant production are covered in depth in Chapters 5 and 57 . Term or late preterm infants with severe RDS at birth that does not have a persistent response to surfactant therapy may need to be evaluated for these mutations.
Many factors influence lung maturation and surfactant production and thus the development of RDS. Both fetal cortisol and thyroxine can stimulate lung maturation. Conversely, insulin delays the maturation of alveolar type II cells, decreasing the proportion of saturated PC, and inhibits the production of SP-A and SP-B. Infants of diabetic mothers also have delayed appearance of PG, and the infant’s L:S ratio is less predictive of lung maturation. The increased incidence of RDS in males (1.7 : 1) may be due to the increased levels of fetal androgens. Fetal androgens delay lung maturation and PG production (by approximately a week) through direct action on lung fibroblasts. Any factors that affect the integrity of the alveolar capillary membrane (asphyxia, hypothermia, or hypoxia) will lead to increased airspace protein and deactivation of surfactant function. Although often necessary in the resuscitation of preterm infants, PPV can release proteins and cause inflammation that worsens RDS. Increased intrauterine inflammation from chorioamnionitis matures the lung and decreases the risk of RDS but may increase the risk of BPD.
Clinical Presentation
Infants with RDS typically present within 4 hours of birth with tachypnea (respiratory rate > 60 breaths/min), intercostal and subcostal retraction, nasal flaring, and abdominal breathing. Although severe cases of RDS present with cyanosis, milder cases of RDS are discovered by routine use of pulse oximetry in infants unable to maintain their saturations greater than 90 on RA. The high surface tension in the alveoli created by the surfactant deficiency and the weaker chest wall muscles of the preterm infant may make it difficult for the infant to develop the normal FRC of 25–30 mL/kg, and thus the infant’s lungs remain in a partially deflated state. The infant may attempt to increase their FRC through an expiratory grunt, created when the infant simultaneously contracts the diaphragm and the constrictor muscles of the larynx to close the upper airway. The resultant exhalation of air is exaggerated and creates the grunting sound found in RDS. The diffuse collapse of the alveoli and smaller airways creates the classic chest radiograph findings of ground-glass appearance with air bronchograms. In infants who are not treated with exogenous surfactant, the symptoms worsen over the first 24–36 hours due to collapse of alveoli recruited by the initial breaths at birth and the deactivation of the small amounts of surfactant present in an infant with RDS. The ongoing lung injury from atelectasis and poor ventilation leads to further leak of inhibitory plasma proteins into the airspace, a cycle that can be prevented by the treatment with exogenous surfactant or CPAP. Without antenatal steroids, endogenous levels of surfactant begin to increase in the airspaces by 24 hours after birth. Between 36 and 48 hours of age, the endogenous production of surfactant is sufficient to maintain alveolar patency and clinical symptoms improve. Often this process is associated with a spontaneous diuresis. With eventual development of surfactant production, recovery from RDS with decreasing oxygen requirements normally occurs by approximately 1 week of age. Extremely preterm infants often require ongoing ventilator support due to chest wall weakness and central respiratory drive issues. The introduction of exogenous surfactant treatment and antenatal steroids has altered the traditional clinical progression over a course of days because most larger infants with significant respiratory distress will receive surfactant and this will be recycled by their type II cells multiple times prior to their own endogenous production.
Diagnosis and Differential Diagnosis
The diagnosis of RDS is made on clinical history (described previously) and chest radiographic appearance. The chest radiograph shows fine granular opacification in both lung fields due to diffuse atelectasis and air bronchograms, where the air-filled bronchi stand out against the atelectatic lungs ( Fig. 19.2 ). In severe cases the lungs are collapsed to the extent that the cardiac silhouette is indistinguishable from the lungs. If the radiograph is taken during the first 4 hours, the retention of fetal lung fluid can make interpretation difficult. As the fluid clears, the chest radiograph appearance may show marked improvement. It should be noted that an infant on mechanical ventilation with adequate tidal volume ventilation and PEEP will have a moderately clear x-ray, even in the setting of significant surfactant deficiency. This difference can be determined clinically because the infants with surfactant deficiency will require higher pressures to maintain tidal volumes. Preterm infants with low lung compliance (i.e., requiring peak inspiratory pressure [PIP] greater than approximately 20 cm H 2 O to generate a tidal volume of 5 mL/kg) would benefit from exogenous surfactant therapy. Although measurement of surfactant in a tracheal aspirate can be performed, this is currently not clinically available. Tracheal secretions or gastric contents can be aspirated and a bubble shake test can be performed to give a basic assessment of surfactant levels, but this is rarely done in the NICU.

Fetal lung fluid normally moves up the trachea and is swallowed by the fetus, but some of the fluid mixes with the amniotic fluid, allowing surfactant to be measured. As the lung matures, the amount of dipalmitoylphosphatidylcholine (DPPC) in the amniotic fluid increases, but the amount of sphingomyelin remains unchanged throughout gestation; thus lung maturity can be assessed from the L:S ratio. The lower the L:S ratio, the more likely that the infant will develop RDS: 80% of infants with a L:S ratio < 1.5 have RDS, compared with 21% of infants with a ratio of 1.5–2.0, and approximately 5% if L:S ratio > 2.0 (usually is associated with lung maturity). Because of the abnormalities in PG formation (not measured in L:S ratio) in infants of diabetic mothers and infants with severe hemolytic disease, these infants may have a mature L:S ratio but have significant RDS. L:S ratios can be altered by the presence of blood, meconium, or vaginal secretions in the sample. Lung maturity can also be assessed by the lamellar body count in the amniotic fluid. Lamellar body counts are more sensitive than L:S ratio and >37,000 correlates well with lung maturity, even in diabetic patients (30,000 in nondiabetic women). Lamellar body counts can be easily obtained by running amniotic fluid through an automated hematology analyzer because they are the same size as platelets. Some investigators suggest that examining lamellar body counts in gastric aspirates of preterm infants may be helpful to guide the early use of surfactant.
It is nearly impossible to differentiate severe early-onset sepsis or pneumonia from RDS, either clinically or radiographically. Because these conditions can also coexist, infants experiencing respiratory distress at birth should have an appropriate workup performed for bacterial infections (blood cultures, complete blood count [CBC], and C-reactive protein at >4 hours) and should then be started on antibiotics. Clinicians may consider holding antibiotics in the setting of preterm delivery for maternal reasons (preeclampsia, Hemolysis, Elevated Liver enzymes, and Low Platelet count [HELLP] syndrome). Antibiotics should be stopped after blood cultures and other lab results are negative. Ampicillin and gentamicin act synergistically against group B streptococcus (GBS) and are also effective against many organisms that cause early-onset septicemia and pneumonia. It is important to know the local antibiotic resistance patterns as gentamicin resistant gram-negative bacteria have become more prevalent. Infants with high oxygen requirements, but a clear chest x-ray or low lung compliance, may be suffering from pulmonary hypertension. This can often be diagnosed with preductal and postductal pulse-oximetry or echocardiography and can be treated with sedation and inhaled nitric oxide (iNO). TTN (discussed later) can present with similar findings to RDS but typically improves more quickly as the excessive water in the lungs is absorbed and the function of the (already present) surfactant improves. Respiratory distress that presents after 4–6 hours of age is usually due to pneumonia; the differential diagnosis includes air leak, heart failure secondary to congenital heart disease, and aspiration.
Management
The appropriate management of preterm infants with RDS has been studied extensively over the past decade, yet there is not a clear consensus among neonatologists about the best approach to take in the delivery room. Everyone would probably agree that RDS is due to a combination of chest wall immaturity and surfactant deficiency and that all treatment methods are merely approaches to bridge the time until the infant begins to develop sufficient endogenous surfactant (typically over 48 hours) and grows stronger to maintain adequate respiration (days to weeks). The three typical management styles for RDS (described in detail later) all derive from these principles: (1) use of CPAP to maintain alveolar distention during spontaneous breathing, (2) giving exogenous surfactant while the infant is spontaneously breathing (minimally invasive surfactant therapy [MIST] or less invasive surfactant therapy [LIST]), or (3) mechanical ventilation and administration of surfactant therapy, with rapid extubation as part of INSURE (Intubation, Surfactant, Rapid Extubation) protocols. Other forms of noninvasive respiratory support (high-flow nasal cannula and NIPPV) have also been tried for RDS.
Since its introduction in the early 1990s, exogenous surfactant therapy has had a dramatic effect on the survival and long-term morbidity from RDS. The first description of surfactant deficiency was published by Avery in 1959, but it took nearly 30 years to get an animal-extracted surfactant approved for clinical use in premature infants. Surfactant therapy, when given as “rescue therapy” in infants with established RDS, decreased the rates of pneumothorax, mortality, and the combined outcome of mortality and BPD. Surfactant therapy has also been shown to be more effective if given as “prophylactic therapy” to infants born less than 28 weeks gestation versus given as rescue therapy, with decreased risk of pneumothorax, pulmonary interstitial emphysema (PIE), and mortality or BPD. It should be noted that the majority of the surfactant therapeutic trials were conducted prior to the ACOG recommendation for antenatal steroid use in 1994 and the infants included in the studies may be slightly less mature than extremely preterm infants who have received steroids. The benefit of prophylactic surfactant in the setting of antenatal steroids has been addressed by multiple trials of surfactant versus CPAP (discussed later). Most intubated extremely preterm infants, even in the setting of intubation for respiratory drive or apnea, would benefit from surfactant therapy because mechanical ventilation alone may release proteins that inhibit the endogenous surfactant. Benefits are shown after a single dose of 100 mg/kg surfactant, but most studies demonstrate that better results are obtained with more than one dose. The results of additional surfactant begin to diminish after the second dose of natural surfactants. There may be differences between bovine surfactant (beractant) and porcine surfactant (poractant alfa), with further benefits on BPD, mortality, and patent ductus arteriosus (PDA) seen with poractant. These differences between surfactants may be due to the higher dosing concentrations given with poractant (200 mg/kg) versus the beractant (100 mg/kg). Natural (animal-derived) surfactants when compared with synthetic surfactant (protein free) are associated with a significant reduction in mortality and pneumothorax but may be associated with an increased risk of NEC. The most well-studied protein-free surfactant, colfosceril palmitate (Exosurf), is no longer being produced. Synthetic surfactants are now available that contain synthetic polypeptide proteins designed to mimic surfactant proteins B or C. The most studied polypeptide is KL4, designed to mimic the C-terminal end of surfactant protein B, which when mixed with DPPC, POPG phospholipids, and palmitic acid is called lucinactant (Surfaxin). KL4 appears to be more resistant to the inhibitory effects of proteins than natural surfactants. Transient hypoxemia and bradycardia may be present during surfactant treatment via ET tube. A transient perturbation in cerebral hemodynamics is also present but is not associated with increased risk of cerebral hemorrhage. Pulmonary hemorrhage has been noted following surfactant administration. Surfactant, once instilled into the trachea or bronchus, will quickly spread throughout the lungs with each breath. Although surfactant dosing is approved for four quadrants, most clinicians instill surfactant with only two positions. In some surfactant-deficient preterm infants, the ET tube serves mainly as a conduit for administering surfactant, but intubation may expose the infant to injurious ventilation and cardiovascular changes. To avoid continued ventilation after surfactant treatment, the INSURE technique has been adopted by many neonatologists, especially for more mature infants (>28 weeks GA) in need of surfactant treatment. To avoid intubation in spontaneously breathing infants on CPAP with higher oxygen needs, surfactant can be given through a thin catheter into the trachea of extremely preterm infants. MIST or LIST involve direct visualization of the vocal cords by direct laryngoscopy and insertion of a feeding tube into the upper trachea ( www.youtube.com/watch?v=OUvgJ57FQR8 ). Surfactant is then quickly instilled through the feeding tube. MIST therapy has been shown to be effective and may avoid unnecessary mechanical ventilation in preterm infants. Surfactant can also be given through a laryngeal mask airway (LMA), but LMAs small enough for extremely preterm infants are still in development. Nebulization of surfactant in spontaneously breathing infants on CPAP is being studied in clinical trials. It is known that some extremely preterm infants are surfactant deficient and need surfactant treatment, but the best way to deliver it is still being determined.
Not all infants with clinical signs of RDS are surfactant deficient, especially in the setting of greater than 48 hours of antenatal steroids, and many of these infants require only CPAP to support the weak respiratory muscles and to stimulate breathing. The mildest forms of RDS can sometimes be managed with supplemental oxygen, but most babies need some form of distending pressure to prevent atelectasis and improve oxygenation. Many centers are trialing all infants on CPAP in the delivery room, and approximately 50% of infants born less than 750 g can be maintained on only CPAP, with much higher rates in more mature infants. The ability to be maintained on CPAP is likely due to the small amount of endogenous surfactant (>5% of term surfactant pool size needed in preterm sheep) necessary to maintain alveolar expansion. Over the past decade, during an era of greater than 80% antenatal steroid rates, five major trials (Continuous Positive Airway Pressure or Intubation at Birth trial [COIN]; Surfactant, Positive Pressure, and Oxygenation Randomized Trial [SUPPORT]; Vermont-Oxford Network [VON] delivery room study; an international randomized controlled trial to evaluate the efficacy of combining prophylactic surfactant and early nasal continuous positive airway pressure in very preterm infants [CUPAP]; and Neocosur Network) have been conducted to compare CPAP versus intubation and surfactant in extremely preterm infants less than 29 weeks. Even with the use of early CPAP in the delivery room, the decrease in the incidence of BPD is modest at approximately 10%. The increased rate of pneumothorax found in the COIN trial was not found in any of the other clinical trials, with an overall pneumothorax rate in all trials of CPAP 6.3% versus surfactant 5.8%. Based on these studies, the 2015 ILCOR resuscitation guidelines recommend treating spontaneously breathing preterm infants (<30 weeks) with CPAP instead of intubation. Preterm infants started on CPAP may initially do well (FiO 2 less than 30%), and then having increasing oxygen requirements and hypercapnia as alveoli begin to collapse. Although PcCO 2 > 60 mm Hg and a FiO 2 > 0.6 is used by some centers as criteria for CPAP failure (including some centers with very low BPD rates), 84% of preterm infants on CPAP with a FiO 2 of >0.4 will reach >0.6. Earlier rescue surfactant, given when FiO 2 < 0.45, has been shown to be beneficial for air leaks, and it is our practice to intubate these infants and give them surfactant if they require FiO 2 > 0.4 on high levels of CPAP. Increasing the level of CPAP increases the lung volume and hence improves oxygenation, but it is possible to cause overdistension, carbon dioxide retention, and air leaks. CPAP can be generated by a mechanical ventilator, a T-piece resuscitator, or an underwater seal (bubble) device (bubble continuous positive airway pressure [BCPAP]). BCPAP is attractive, especially for low-resource countries, because the CPAP is regulated by submerging the expiratory limb of the gas circuit a set distance under water (actual cm H 2 O). It should be noted that the distending pressure generated by BCPAP is flow dependent and intraprong pressures may be higher (up to 1.3 cm H 2 O) than expected for the depth of submersion in water. Unlike ventilator-derived CPAP, BCPAP generates a variable distending pressure, often referred to as the “noise” of BCPAP. In intubated sheep, BCPAP had improved gas exchange, oxygenation, and pH compared with ventilator-derived CPAP, and similar benefit was seen on oxygenation in preterm infants on BCPAP. When the pressure fluctuations are further increased (high-amplitude BCPAP), the oscillatory pressure wave can achieve similar blood gas values in paralyzed, saline-lavaged rabbits to conventional mechanical ventilation. The transmission of the oscillatory bubbles and the CPAP pressure may differ based on the nasal interface used, with the RAM cannula losing more CPAP pressure than other nasal-prong interfaces. Heated humidified high-flow nasal cannula (HHHFNC) is used by some units to treat RDS and has been shown not to be “inferior” to CPAP in clinical trials. The variability of pressures generated in preterm infants on HHHFNC, often caused by leaks through the mouth, makes it a less predictable form of distending pressure.
Preterm infants may need PPV due to poor respiratory drive (apnea of prematurity), inability to maintain chest expansion on CPAP (surfactant deficiency, respiratory muscle weakness), or ineffective gas exchange (hypoxia or hypercarbia). PPV can be given via an ET tube (traditional mechanical ventilation) or through nasal prongs (NIPPV). In meta-analysis of studies comparing NIPPV with CPAP following extubation, NIPPV reduced the incidence of extubation failure but had no effect on BPD or mortality. Although earlier studies demonstrated a benefit of synchronized NIPPV on BPD, more recent, larger studies, which used unsynchronized NIPPV with lower average PIPs, found no difference. In unsynchronized NIPPV, only 25% of breaths were coordinated with infant’s breaths and only these breaths led to an increase tidal volume. The ability to synchronize breaths can now be done with neurally adjusted ventilatory assist (NAVA), which uses an esophageal catheter to measure diaphragmatic electrical activity to both synchronize breaths and adjust ventilator pressures. NAVA has been shown to decrease BPD in some small studies but needs to be studied in a randomized trial for prevention of BPD. Conventional mechanical ventilators deliver intermittent positive-pressure inflations and PEEP at a preset rate and inspiratory time. The ventilator inflations are often not synchronized with the infant’s breaths, and this can contribute to air leak syndromes. Many neonatologists now use higher rates and lower tidal volumes to improve asynchrony and decrease possible lung injury from alveolar overdistention. Volutrauma, from high tidal volumes, and atelectrauma, from inadequate stenting of the airways with PEEP at the end of expiration, are likely more dangerous to the developing lung than the pressures used to generate these volumes. The development of infant mechanical ventilators with accurate flow sensors at the end of the ET tube has allowed for the more reliable use of volume ventilation in the NICU. Studies comparing volume to pressure ventilation in preterm infants demonstrate a benefit for volume ventilation of reduced death or BPD, duration of ventilation, pneumothoraces, periventricular leukomalacia, and severe intraventricular hemorrhage. Volume ventilation also allows for rapid changes in inspiratory pressures in response to changes in lung compliance, especially in the setting of exogenous surfactant administration or use of postnatal corticosteroids. An alternative approach to conventional mechanical ventilation, which generates a tidal volume of 4–6 mL/kg through either pressure or volume control, is to use extremely small volumes but very high rates. During high-frequency jet ventilation (HFJV), high-velocity bursts of gas are fired at rates of 200–600 per minute to generate gas flow down the ET tube. There are few randomized trials comparing HFJV with conventional ventilation, but the largest included approximately 130 infants and demonstrated a slight decrease in BPD rates and need for home oxygen. High-frequency oscillatory ventilation (HFOV) uses a piston to generate a gas gradient within the dead space of the trachea, with gas exchange reliant on oxygen moving down the gradient into the lungs and carbon dioxide diffusing along its gradient out of the lung. Because HFOV relies on forming oxygen and carbon dioxide gradients, extremely active infants or those breathing against the ventilator may affect the gas exchange ability of the ventilator. Randomized trials comparing HFOV to conventional ventilation have variable results, but a meta-analysis demonstrated that HFOV is associated with a modest reduction in BPD, with no excess of intraventricular hemorrhage (IVH) or periventricular leukomalacia (PVL). Surprisingly, especially because many clinicians use HFOV in setting of PIE, HFOV was also associated with a mild increase in air leaks. Use of open lung strategies with HFOV, where mean airway pressure (MAP) is increased until there is no longer an improvement in oxygenation and then decreased by 2 cm H 2 O, may lead to improved pulmonary responses in preterm infants. Infants with RDS whose hypoxia is more severe than would be anticipated from the chest radiograph, and who have low lung compliance, should be evaluated for pulmonary hypertension.
Preventative Strategies
Antenatal steroids (dexamethasone or betamethasone) can cross the placenta to mature the fetal lung and brain. In the lung, antenatal steroids can decrease the fetal lung fluid through activation of ENaCs, induce the production of surfactant proteins and lipid synthesis, and alter preterm responses to oxidative stress. Randomized trials have demonstrated that administration of antenatal corticosteroids significantly reduces the incidences of RDS, neonatal death, cerebral hemorrhage, and NEC. Guidelines recommend the routine use of antenatal steroids for mothers at risk for preterm delivery from 24 weeks to 34 weeks GA. Betamethasone (two doses 24 hours apart), rather than dexamethasone (four doses 12 hours apart), is preferred. Antenatal steroids can be considered at 23 weeks GA but are not currently recommended at 22 weeks GA. Studies have also demonstrated decreased respiratory complications in late preterm infants (34–36 weeks) randomized to antenatal corticosteroids, and revisions to ACOG guidelines are underway. Studies of antenatal steroids prior to elective C-section also demonstrate some benefit on respiratory symptoms at birth. The benefit of steroids is maximal in infants delivered between 24 and 168 hours after maternal therapy, but benefits on the lungs are seen in less than 24 hours. The use of repetitive courses of antenatal steroids showed some benefits for respiratory outcomes, but more than five weekly courses caused decreased fetal growth and head circumference. Infants who received repetitive dosing did not show differences in adverse neurologic outcomes at 18-month follow-up. Some obstetricians will give an additional dose of steroids to a mother with persistent threat of preterm delivery 1–2 weeks after initial course. Antenatal steroids do not increase the risk of infection in pregnancies complicated by preterm prelabor rupture of the membranes. There is not a consensus opinion about the use of antenatal steroids for infants of mothers with possible chorioamnionitis.
Thyroid hormones induce surfactant synthesis in animal models. Randomized trials of antenatal administration of thyrotropin-releasing hormone (the only component of the pathway that crosses the placenta) did not reduce the risk of neonatal respiratory distress or BPD, and unfortunately increased the risk of lower 5-minute Apgar scores and caused transient suppression of the pituitary system. Other drugs such as aminophylline, ambroxol, and terbutaline have also been tried, with variable success.
Mortality and Morbidity
Overall the mortality from RDS is 5%–10%; the mortality rate is inversely proportional to GA. The use of antenatal steroids and exogenous surfactant therapy has decreased the mortality from RDS, but significant morbidities still exist in the extremely preterm infants that survive. The acute complications of RDS include air leaks, PDA, and pulmonary hemorrhage (discussed separately later). BPD, defined as either oxygen dependency 28 days after birth or at 36 weeks corrected GA, develops in more than 40% of infants born prior to 29 weeks of gestation. BPD is discussed at length in Chapter 20 . Some of these infants require tracheostomy and prolonged mechanical ventilation (see Chapter 21 ). Changes in antenatal steroid use, modes of respiratory support, and endogenous surfactant have fortunately created a very different form of RDS than was first described by Northway in 1967.
Transient Tachypnea of the Newborn
Epidemiology
Although the exact numbers are unclear, TTN occurs in less than 1% of term vaginal deliveries but is more frequent in late preterm infants. Each week of GA after 35 weeks reduces the risk of respiratory symptoms, particularly if primary C-section is performed. Other risk factors for TTN include C-section birth, with or without labor (threefold higher risk), male sex, macrosomia, maternal diabetes, and maternal history of asthma. Infants of asthmatic mothers may have a genetic predisposition to decreased responsiveness of β-adrenergic stimulation of ENaCs, increasing the risk of TTN and RDS. Antenatal corticosteroids given to women at risk for preterm labor at 34–36 weeks decreased the risk of respiratory support and oxygen need.
Pathophysiology
This syndrome is thought to result directly from ineffective clearance of fetal lung fluid at the time of birth (see previous description of lung clearance). The lack of uterine contractions causing spinal flexion, and catecholamine surge from labor, lead to increased fetal lung fluid at birth and an increased risk of TTN with elective C-section. The excessive fluid in the lung interacts with the surfactant, which is often at adequate levels to maintain alveolar expansion, and leads to relative inactivation of the surfactant until the fluid is absorbed. A subpopulation of term infants with TTN, often ones who have prolonged hypoxia and tachypnea, have a degree of surfactant deficiency that contributes to the respiratory distress. Inadequate Na + transport out of the airways, either because of decreased numbers of ENaCs or lack of activation of these channels, contributes to the excess fluid. The number of water channels (aquaporins) may also differ in infants with TTN. Preterm infants also have decreased Na + transport, and late preterm infants with TTN have low amounts of surfactant. The increased fluid accumulates in the interstitial space generating increased pressure and increased refilling of the airways with fluid during expiration, which can be overcome by CPAP in many infants.
Clinical Presentation
Infants with TTN are tachypneic with respiratory rates that can be greater than 80 breaths/min. The clinical presentation can resemble mild RDS, although the infants have grunting less often. The chest may be barrel shaped because of hyperinflation. The chest radiograph shows hyperinflation, prominent perihilar vascular markings due to engorgement of the periarterial lymphatics, edema of the interlobar septae, and fluid in the fissures ( Fig. 19.3 ). Some infants with TTN will develop pulmonary hypertension, and this should be suspected in infants requiring high concentrations of oxygen.

Management
Most infants with TTN can be managed with supplemental oxygen or CPAP. CPAP will help to drive the excessive fluid out of airways and into the interstitial spaces, where it is absorbed over a few hours. Except in the setting of elective C-section or C-section for maternal reasons not related to infection, IV antimicrobials should be considered because sepsis and pneumonia in the term infant can mimic TTN clinically. TTN is usually self-limiting, and affected infants usually have significant clinical improvement within the first 24 hours and complete recovery within a few days of birth. Complications are rare, but air leaks may occur. Many infants with TTN have respiratory rates too high for safe oral feeding, so intravenous fluids or gavage feeds may be necessary until the tachypnea improves. Trials of furosemide or racemic epinephrine to increase fluid clearance have not shown clear benefits. More recently, it has been suggested that affected infants may derive clinical benefit from inhaled β-adrenergic agonist therapy ; however, larger trials are required.
Meconium Aspiration Syndrome
Epidemiology
Meconium-stained amniotic fluid (MSAF) complicates approximately 13% of live births, affecting term more than preterm infants. MSAF occurs in less than 5% of preterm pregnancies, and when it does occur it suggests infection. Five percent of babies born through MSAF will develop meconium aspiration syndrome (MAS). In a large study of perinatal registry data in a developed setting, it was found that the overall incidence of MAS was 0.43/1000 live births. The incidence has been decreasing with time; it is associated with factors including advanced GA, low Apgar scores, and C-section delivery. MAS is therefore a condition that predominantly affects term and postterm babies.
Pathophysiology
MASF occurs when there is antenatal passage of meconium. This phenomenon has been associated with fetal distress and hypoxic-ischemic insult. MAS is an inflammatory lung condition caused by aspiration of MSAF by the infant into the airways during the peripartum period.
It has been found that antenatal passage of meconium is associated with higher levels of motilin in the baby. Motilin is produced mainly by endocrine M cells of the duodenojejunal mucosa and stimulates peristalsis. Levels are very low in preterm infants and nonasphyxiated term infants but are raised in asphyxiated term babies who pass meconium during the peripartum period. Prolonged severe fetal hypoxia can stimulate fetal gasping in utero leading to inhalation of MSAF. It is also thought that inhalation can occur perinatally with the first breaths of the baby who is delivered through MSAF. Although meconium is mainly made up of water (approximately 80%), it is chemically complex, containing components of various substances, including digestive juices, lipids, intestinal epithelial cells, lanugo hair, bile, and amniotic fluid. It is of variable consistency and, when inhaled, can create a ball-valve mechanism within the airways. This obstructive pathology predisposes the lungs to air trapping and hyperinflation. In addition, meconium irritates the lungs, activating numerous inflammatory mediators, toll-like receptors, and the complement system. An inflammatory pneumonitis and systemic inflammatory response can ensue, with an associated release of vasoactive substances that can cause vasoconstriction and raised pulmonary arterial pressure. In addition, meconium impairs surfactant function, increasing the possibility of atelectasis. Meconium harbors microbiota that are associated with amniotic fluid and placental microbiota. In addition, meconium is thought to enhance bacterial growth by serving as a growth factor and inhibiting bacteriostatic properties of amniotic fluid, facilitating the growth of pathogens such as Escherichia coli. Meconium has been suggested to significantly impair mechanisms of intracellular microbial killing, inhibiting phagocytosis and the neutrophil oxidative burst.
Clinical Features
Infants with MAS generally present with signs of respiratory distress such as tachypnea and use of accessory muscles of respiration. The obstructive pathology can lead to increased anteroposterior diameter of the chest, whereas the ventilation-perfusion mismatch and pulmonary hypertension can lead to cyanosis. Initial chest radiographs may show hyperinflated lung fields and widespread patchy infiltrates ( Fig. 19.4 ). Small pleural effusions occur in approximately 20% of patients. With development of pneumonitis and interstitial edema the radiographic appearance can progress to diffuse, homogeneous opacification of both lung fields. Air leaks, such as pneumothorax and pneumomediastinum, are very common, occurring in approximately 20% of infants. In those with pulmonary hypertension, right-to-left shunting at ductal and atrial levels may be seen by echocardiography. Differential diagnoses include pneumonia, surfactant deficiency, persistent pulmonary hypertension of the newborn (PPHN), congenital cardiac disease, and aspiration of blood.

Management
Although infants mildly affected by MAS may require no specific treatment, a substantial proportion of infants will require intensive care management of the many complications that can arise. The risk of pulmonary hypertension is high in infants with MAS, and hypoxemia should be avoided. Supplemental oxygen should be administered to keep oxygen saturations greater than 94%. One should also consider using PaO 2 to guide oxygen use. With increasing evidence that high oxygen concentrations can cause harm, ventilatory support measures such as CPAP or mechanical ventilation should be considered if the FiO 2 requirement is persistently high. Traditionally MAS has been conceptualized as a primarily obstructive condition; however, it is now increasingly recognized that areas of hyperinflation may coexist with atelectasis in a setting of ventilation-perfusion mismatch. It is therefore essential that ventilatory support should be initiated with close monitoring of the progress of the infant. Use of CPAP or mechanical ventilation may improve oxygenation but may also increase the risk of air leak. The thresholds at which ventilatory support should be initiated are therefore not evidence based and may need to be adjusted depending on the condition of the individual baby. In general, mechanical ventilation would be considered in infants with PaCO 2 > 8 kPa (>60 mm Hg) and PaO 2 < 6 kPa (<50 mm Hg) despite initial oxygen supplementation, especially in infants with evidence of PPHN. Conventionally, the ventilator strategy should be based on a relatively low PEEP and long expiratory time, but in practice, the appropriateness of this strategy will depend on the relative balance between atelectasis and hyperinflation. In infants with severe disease, and particularly if associated with pulmonary hypertension, HFOV and adjunctive use of inhaled NO should be considered. In a meta-analysis that included four randomized trials, it was suggested that surfactant administration could reduce the severity of respiratory illness and decrease the number of infants with progressive respiratory failure requiring support with extracorporeal membrane oxygenation (ECMO), especially if administered as a bolus. Surfactant administration both as lung lavage and as a bolus reduced duration of mechanical ventilation and length of hospital stay but did not reduce mortality. The use of antibiotics has not been shown to benefit infants with MAS in terms of duration of ventilation, hospital stay, or mortality. However, despite this, it is widespread practice to commence broad-spectrum antibiotics empirically in view of the difficulty in differentiating between MAS and pneumonia. Results of a randomized trial suggested that ECMO improved survival in infants with an oxygenation index (OI) of >40. However, with improving intensive care strategies, the need for ECMO has been reduced. There has been interest in the protective effects of hypothermia for lung conditions such as MAS, and some centers have started to incorporate it into the management of these infants.
Prevention
There is an inverse association between amniotic fluid volume and fetal HR decelerations, possibly due to either cord or head compression. Amnioinfusion has been thought to dilute meconium, especially in cases of thick MSAF, and correct oligohydramnios, thereby relieving umbilical cord compression. However, results of a meta-analysis suggested that amnioinfusion did not improve perinatal outcomes in settings of standard peripartum surveillance and was only beneficial in settings with limited facilities to monitor the baby during labor. Furthermore, amnioinfusion carries increased risk of several adverse outcomes, including cord prolapse, infection, and requirement for instrumental delivery. There has been much debate in the value of meticulous clearing of the airway during and after delivery in an infant delivered through MSAF, with recommendations being based more on biologic plausibility and expert opinion than a strong body of evidence. Avoidance of postterm delivery is the key factor in reducing the incidence of MSAF and thus MAS. With increasing numbers of studies and meta-analyses, there has been a failure to obtain evidence that routine efforts to clear the airway of meconium by ET or oropharyngeal suctioning can prevent MAS or lead to improved outcomes of affected infants. It has been argued that MAS incidence in these studies was low. However, even in a setting with a high incidence of MAS, intrapartum suctioning was not shown to be of benefit. In another recent study in a developing setting, ET suctioning was not shown to be superior to routine resuscitation even for nonvigorous babies delivered through MSAF. Because of the accumulating evidence of lack of benefit of routine suctioning to prevent MAS, ILCOR no longer recommends routine ET suctioning of nonvigorous babies delivered through MSAF. Clinical judgement regarding the presence of significant airway obstruction should be exercised when deciding whether to initiate ET suctioning, taking care not to delay PPV and other important resuscitation measures in the process.
Prognosis
The mortality of MAS in a developed setting has been reported to be 2.5%. In contrast, mortality can be as high as 32% in developing regions of the world. Most deaths are from respiratory failure, pulmonary hypertension, or air leaks. Fifty percent of babies who require mechanical ventilation because of MAS suffer an air leak. Neurodevelopmental delays have been observed even in infants who respond well to conventional ventilation. Children with a history of MAS have been found to exhibit long-term lung function abnormalities, increased bronchial hyperreactivity, and higher reported rates of recurrent cough and wheeze.
Acute Respiratory Distress Syndrome
Pathophysiology
Acute respiratory distress syndrome (ARDS) in the newborn occurs when a systemic injury leads to lung inflammation and injury in previously healthy lungs and is often associated with multiorgan failure. Like adults, ARDS can occur following asphyxia, shock (cardiogenic or hypovolemic), or sepsis. Myocardial dysfunction from birth asphyxia or from severe metabolic acidosis can lead to pulmonary edema and need for ventilator support. The direct injury from the hypoxia on the lung tissue or the injury sustained from mechanical ventilation leads to release of proteins into the air spaces, thus worsening the lung disease by inactivation of surfactant. Sepsis will lead to increased capillary leak of fluid and protein into the lungs. Preterm infants may be predisposed to lung inflammation from chorioamnionitis, and severe chorioamnionitis may have systemic responses—fetal inflammatory response syndrome (FIRS).
Clinical Presentation
ARDS presents with worsening tachypnea and oxygen requirements after a systemic event. Clinically it may look very like primary lung diseases such as pneumonia or MAS (discussed in other sections of this chapter). Some infants who have the clinical appearance of RDS, but who are not premature and do not have a good response to exogenous surfactant therapy, may have underlying lung disease more consistent with ARDS. The tachypnea may result initially from stimulation by metabolic acidosis or damage to the central nervous system, but the hypoxia will progressively worsen as the lung tissue becomes edematous and inflammation develops. The chest radiograph demonstrates diffuse pulmonary infiltrates, with some severe cases having complete opacification of the lungs. Blood cultures and tracheal aspirates may guide the antibiotic management of these infants.
Management
Treatment of the underlying cause of the ARDS (sepsis, birth asphyxia, cardiogenic shock) is essential for the lungs to recover. The newborn lung is moderately resistant to chronic injury and should be able to recover if the infant can survive the initial insult. Surfactant administration can improve oxygenation in ARDS, similar to MAS, but may require larger doses than used in RDS. Efforts to increase the mean airway pressure, through either higher PEEP or longer inspiratory time, will help to increase FRC and improve oxygenation. HFOV, especially when using a series of recruitment steps to reach an open lung, may have benefits for improving oxygenation in ARDS though its superiority over conventional ventilation has not been demonstrated. In recent studies in adults with ARDS, HFOV did not decrease mortality over conventional mechanical ventilation. Fluid management is important in treating ARDS as the clinician tries to decrease flooding of the lung with fluid restriction, while still maintaining proper perfusion of other organs. Broad-spectrum antimicrobials (usually ampicillin plus an aminoglycoside if in the first few days after birth but with broader coverage, especially for staphylococcus, when systemic infection is suspected after 3 days) should be administered. Aminoglycoside levels must be carefully monitored because these infants are at high risk of renal dysfunction. Air leaks and infection are commonly seen in infants with ARDS. Pulmonary hypertension can occur in ARDS and infants may benefit from sedation or inhaled NO therapy. Severe ARDS that does not respond to HFOV may require ECMO, often arteriovenous due to cardiac dysfunction, until the lungs recover. The mortality and morbidity of ARDS are high, and this is due to a combination of the hypoxia from the lung disease and the effects of the systemic disease that caused the ARDS.
Early-Onset Pneumonia
Early-onset pneumonia presents clinically within the first 48 hours to 1 week after birth. Because early-onset or congenital pneumonias are often present at birth, it is sometimes difficult to distinguish pneumonia from RDS, TTN, or MAS. Radiographically diagnosed pneumonia was found in 1.5% of infants born at 34 weeks versus 0.2% of infants born at term. Pneumonias can be acquired either transplacentally from mother, during labor with prolonged rupture of membranes or chorioamnionitis, or during delivery. The timing of clinical presentation will depend on the mode of transmission. The term newborn lung is immature with respect to ciliary clearance of microbes, lung macrophage function, and humoral immune responses (immunoglobulin A, surfactant protein A and D); and this predisposes the newborn lung to pneumonias. Although originally thought to be sterile, the normal lung is now known to be colonized with its own microbiome and the interactions between normal and pathologic bacteria may play a role in the development of pneumonia or other respiratory diseases. The mode of delivery affects the respiratory microbiome, with infants delivered vaginally having bacteria closer to the mother’s vaginal secretions, whereas C-section infants have a lung biome closer to maternal skin. Transplacentally acquired organisms associated with pneumonia include Listeria monocytogenes (often found in unpasteurized cheeses), Mycobacterium tuberculosis, Treponema pallidum, rubella virus, cytomegalovirus (CMV), herpes simplex virus (HSV), adenovirus, and influenza type A virus. A high percentage (more than 20%) of stillborn infants or infants that die soon after birth are found to have pneumonia at autopsy. Most cases caused by ascending infection are due to Streptococcus agalactiae (GBS), and antenatal testing for GBS is recommended for all pregnant women. The use of antenatal GBS prophylaxis has decreased the rates of early onset GBS sepsis and pneumonia but has not changed the rates of late-onset disease. There are 10 identifiable subtypes of GBS based on capsular polysaccharide antigens; most neonatal infections are caused by types Ia, II, III, and V, but the pathogenic subtypes differ between countries. The incidence of early-onset GBS sepsis is 0.67 per 1000 live births in the Americas and 0.53 per 1000 in Europe. Countries reporting no use of intrapartum antibiotics have had a 2.2-fold increase in early-onset disease. E. coli is the second most common cause of early-onset neonatal sepsis and pneumonia. A large percentage of E. coli strains that cause early-onset sepsis possess the capsule type K1, which confers antiphagocytic properties and resistance to complement mediated killing; antibiotic resistance to both ampicillin and gentamicin have been increasingly reported in E. coli. Other organisms that cause ascending infection include Haemophilus influenzae, Streptococcus pneumoniae, L. monocytogenes, Klebsiella pneumoniae, Candida albicans, and viruses such as adenovirus, CMV, HSV, and echovirus.
Risk factors for early-onset pneumonia include prolonged rupture of the membranes, premature labor, and colonization of the vagina with GBS or other pathogens. Chorioamnionitis increases the risk of early-onset pneumonia and may predispose the infant to an altered immune/inflammatory response to microorganisms acquired during labor. In developed countries, approximately 20%–30% of women are colonized with GBS in the vaginal vault and 50% of infants born vaginally will become colonized. Fortunately, only 1% of these infants will develop invasive GBS disease. Although not routinely tested clinically, the highest transmission rate is in GBS colonized women who have low levels of circulating anti-GBS immunoglobulin. Women infected with HIV are considerably more susceptible to many perinatal infections, especially L. monocytogenes. HSV is usually transmitted during delivery through an infected maternal genital tract.
Clinical Presentation
The timing of presentation depends on whether the infection was acquired transplacentally, usually present at birth, or during labor, when it may take up to 48 hours to develop. GBS is a fast-growing microorganism, and most of these infections will present in the first 12 hours, and rapid deterioration can occur without prompt treatment. In the setting of chorioamnionitis, sepsis may present within 6 hours of birth. Many infants with pneumonia or sepsis have normal Apgar values at birth but develop progressive tachypnea, respiratory distress, hypoxia, and other signs of sepsis. Some infants will have more subtle findings, such as poor feeding, irritability, hypothermia, or fever. Infants who are overtly septic with poor peripheral perfusion, cyanosis, and inadequate respiration have a poor prognosis. Infants with severe congenital pneumonia may require high PIPs to open the inflamed lungs. Infants with congenitally acquired Listeria infection are often extremely ill at birth, with severe pneumonia and hepatomegaly, and at autopsy have small pinkish-gray granulomas throughout the lung, liver, skin, and other organ systems. Viruses such as CMV and HSV can present with pneumonia or more generalized pneumonitis.
The chest radiograph appearance is variable and can range from an entire lobe ( Fig. 19.5 ) to segmental consolidation, atelectasis, or diffuse opacification ( Fig. 19.6 ). Many pediatric radiologists will not distinguish between neonatal pneumonia, TTN, or RDS, and often a follow-up image is needed (TTN should have resolved on repeat x-ray). Pleural effusions can be present and sometimes abscesses or pneumatoceles. For intubated infants, a tracheal aspirate from a new ET tube can sometimes be useful and should be evaluated by microscopy for white blood cell, and culture. Blood cultures are important because many of the infections are hematogenously spread or have secondary bacterial release into the bloodstream. Systemic markers of infection and inflammation, such as a CBC or C-reactive protein, may help to guide the clinician regarding antibiotic use. In the setting of suspected chorioamnionitis, histologic evaluation and culture of the placenta may provide additional information on the organism. If herpes infection is likely, viral cultures from the maternal lesions and the infant should be obtained.
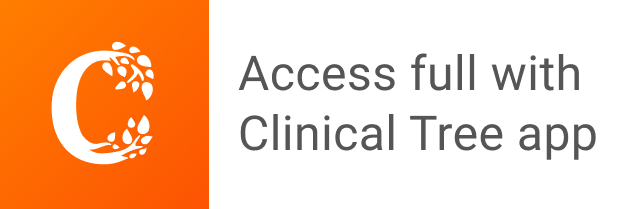