Abstract
A significant number of children admitted to pediatric intensive care units across the United States receive invasive mechanical ventilation. While respiratory disease in children is a major indication for endotracheal intubation and ventilation, these patients comprise only one-fifth of pediatric ICU admissions, and there are numerous nonrespiratory indications for mechanical ventilation, including postoperative care and pain management, neurologic and neuromuscular pathology, congenital heart disease, cardiomyopathy, and shock. However, mechanical ventilation can be associated with significant complications that are less commonly encountered outside the ICU setting. These include mucus plugging, atelectasis, ventilator-induced lung injury, ventilator-associated pneumonia, air leak syndromes, and ICU neuromyopathy. This chapter discusses the risk factors, presentation, and evidence-based management of these ICU-related respiratory complications. Additionally, this chapter discusses special pulmonary considerations in management of critically ill children with congenital heart diseases, neuromuscular diseases, malignancy, and hematopoietic stem cell transplantation.
Keywords
acute respiratory failure, pediatric intensive care unit, mechanical ventilation, endotracheal intubation, high-frequency ventilation, extracorporeal membranous oxygenation, mucus plugging, ventilator-associated pneumonia, ventilator-induced lung injury, pneumothorax, pneumomediastinum, pulmonary hemorrhage, ICU, neuromyopathy, congenital heart disease, bone marrow transplantation
History of Pediatric Intensive Care
The first pediatric intensive care unit (PICU) was established in 1955 at Children’s Hospital of Göteborg in Sweden, followed by the emergence of PICUs in the United States in the late 1950s and early 1960s. These units originated to meet the medical needs of critically ill children who were undergoing increasingly extensive and complicated surgical procedures or recovering from complex medical problems. In these early PICUs, anesthesiologists continued to provide the cardiorespiratory support and pain control that these patients required in the operating room. During the 1970s and 1980s, care teams involving surgeons, anesthesiologists, and pediatric subspecialists evolved, with the leader of that team being the pediatric intensivist. In the early years, these intensivists were largely derived from the ranks of pediatric anesthesiologists with the percentage of pediatricians becoming intensivists steadily increasing through the late 1980s and early 1990s. The Society of Critical Care Medicine (SCCM), which originated as an adult intensive care society, recognized pediatric critical care medicine as a discrete entity and created a separate section of pediatric critical care within the SCCM in 1981. Within 2 years, the section created guidelines defining the minimal requirements for pediatric ICUs. The American Board of Pediatrics sponsored the first pediatric critical care board exam in 1991. Although the majority of pediatric intensivists have completed a pediatrics residency prior to entering the field, many critical care divisions remain within departments of anesthesiology.
Indications for Intubation and Mechanical Ventilation
Mechanical ventilation of acutely ill children remains one of the principal therapies provided in PICUs throughout the United States. Available data from the Pediatric Health Information System (PHIS) database and other sources suggests that 20%–30% of all children admitted to PICUs across the United States receive invasive mechanical ventilation. While respiratory disease in children is a major indication for endotracheal intubation and ventilation, these patients comprise only one-fifth of PICU admissions, and there are numerous nonrespiratory indications for mechanical ventilation, including postoperative care and pain management, neurological and neuromuscular pathology, congenital heart disease, cardiomyopathy, and shock ( Table 37.1 ).
Primary Category | Primary Pathophysiologic Mechanism | Clinical Example |
---|---|---|
Respiratory causes | Restrictive pathology (Decreased lung and/or chest wall compliance, low FRC) | Pneumonia Acute respiratory distress syndrome Atelectasis Large pleural effusion or ascites Severe scoliosis |
Obstructive pathology—upper airway | Croup Epiglottitis | |
Obstructive pathology—lower airway | Bronchiolitis Asthma | |
CNS pathology | Poor or absent respiratory drive | Encephalitis Encephalopathy Ingestion of medications Electrolyte disturbance |
Inability to maintain airway | Poor or absent gag/cough | |
Increased intracranial pressure | Traumatic brain injury | |
Neuromuscular pathology | Severe weakness | Electrolyte disturbance Spinal muscular atrophy Myasthenia gravis Guillain-Barré syndrome Tick paralysis Spinal cord injury |
Weakness with inability to protect airway Diaphragmatic paralysis Flail chest | Infants disproportionately affected | |
Cardiovascular dysfunction | Severe shock Severe myocardial dysfunction |
There has recently been increasing emphasis on the use of noninvasive mechanical ventilation (NIV) in the form of continuous positive airway pressure (CPAP) and bilevel positive airway pressure (BIPAP) to delay or avoid endotracheal intubation and invasive mechanical ventilation. Noninvasive mechanical ventilation is commonly used among adults with cardiogenic pulmonary edema, chronic obstructive pulmonary disease (COPD) exacerbation, and neuromuscular disorders. Among preterm neonates with respiratory distress syndrome, noninvasive CPAP use is common and reduces the need for invasive mechanical ventilation. No clear indications exist for the use of noninvasive modalities as a standard therapy among infants and children in PICUs. However, data suggest that its use is rapidly increasing, and more pediatric ICUs are adopting the use of NIV for managing mild to moderate acute respiratory failure and in children with chronic conditions such as neuromuscular weakness (e.g., Duchenne’s muscular dystrophy and spinal muscular atrophy) or restrictive lung disease. Acute conditions that are transient and self-limiting in nature may be amenable to noninvasive mechanical support and include upper airway obstruction (e.g., croup, postextubation upper airway edema), lower airway obstruction (e.g., asthma, bronchiolitis), and lung parenchymal diseases such as bronchiolitis, pneumonia, or pulmonary edema. NIV does not afford a secure airway, and masks may impede airway clearance in the event of vomiting. NIV should therefore be used with caution in patients with poor neurologic function or with potential for rapid deterioration. Examples include severe trauma, cardiomyopathy, and septic shock. Underlying anatomical or airway anomalies that prevent the proper fitting of noninvasive interfaces also preclude the use of NIV. Similarly, patients with respiratory failure following gastrointestinal tract surgeries or disruption of the integrity of the airway are rarely suitable candidates, as positive pressure could disrupt the surgical sites.
Ventilation Strategies
Conventional Versus Oscillatory Ventilation
PICUs worldwide use a wide variety of ventilation modes. Conventional ventilation modes cycle air or oxygen enriched gas mixtures through the respiratory tract by bulk airflow. Commonly employed modes include pressure control (PC), volume control (VC), or VC modes with decelerating flow that minimizes peak inspiratory pressures. These breaths are cycled using synchronized intermittent mandatory ventilation (SIMV) typically with pressure support for breaths above the set respiratory rate or assist control (AC) in which each breath above that rate is a controlled breath. Most modern ventilators also incorporate one or more support-modes or autoweaning support modes with embedded algorithms to ensure patient safety. Ventilators have traditionally synchronized breaths to patient effort using flow or pressure triggers. A newer innovation, neurally adjusted ventilator assist (NAVA) uses diaphragmatic electrical activity to initiate and terminate breath deliver and modulate flow. Selection of a particular conventional ventilation mode is often not targeted specifically to the underlying disease but rather is determined by the intensive care physician’s experience, local PICU policy and protocols, or outcomes of studies in adults.
Less commonly used and unconventional modes of ventilation include high-frequency ventilation (HFV) and airway pressure release ventilation (APRV). High-frequency oscillatory ventilation (HFOV) is the predominant method of HFV used in the PICU setting, other methods being high-frequency jet ventilation, and high-frequency percussive ventilation (HFPV). High-frequency jet ventilators (HFJVs) contain flow interrupters that disrupt high velocity streams of gas, generating high-pressured jets that deliver small tidal volumes at supraphysiologic frequencies. HFPV delivers a series of high-frequency subtidal volumes in combination with low-frequency breathing cycles. HFPV may be defined as a time-cycled pressure-controlled ventilator coupled with a high-frequency flow generator. Most trials comparing HFV to conventional ventilation only assessed the role of oscillators; therefore, we only discuss the use of HFOV in further detail in this chapter.
During HFOV, airway pressure is maintained by constant gas flow (bias flow) through a valve that can be adjusted to increase or decrease mean airway pressure (MAP). Pressure waves are generated by a bellows that oscillates at supraphysiological ventilatory frequencies between 3 and 15 Hz (180–900 breaths per minute); the pattern of ventilation is akin to panting in dogs. The oscillator creates both inspiratory and expiratory pressure waves, because the bellows is actively driven in both directions. Therefore, expiration is also active, which differentiates HFOV from conventional ventilators, HFJV and HFPV, where expiration is passive and dependent on the elastic recoil of the respiratory system. Active expiration may be beneficial in preventing hyperinflation and enhancing CO 2 elimination. Tidal volumes generated with HFOV are smaller than the anatomic dead space. Therefore, unlike conventional ventilation, which relies principally upon bulk flow of gas to the alveoli, ventilation during HFOV is accomplished largely by enhanced gas mixing within the lung. The numerous mechanisms that account for the ability to achieve adequate ventilation with HFOV are outlined in Fig. 37.1 , and include bulk convection, cardiac oscillations, Taylor dispersion, asymmetric velocity profiles, pendelluft, and diffusion. For a detailed discussion on mechanical ventilation modes, please refer to Chapter 34 , Principles of Mechanical Ventilation.

Use of HFOV in the PICU was largely driven from positive studies on its use in the neonatal intensive care unit (NICU) ; however, recent large randomized control trials in adults have failed to show benefit. Randomized controlled trials evaluating HFOV among children are lacking. A recently published retrospective observational study and a propensity score matched secondary analysis of another pediatric RCT compared early HFOV (initiated within 24–48 hours of intubation) to conventional mechanical ventilation and/or late HFOV. While the studies suggested increased duration of mechanical ventilation and mortality in HFOV groups, patients treated with HFOV also had poorer oxygenation than non-HFOV groups. Thus, the decision as to whether and when to initiate HFOV in the PICU is based more upon clinical experience than empiric evidence.
Extracorporeal Membranous Oxygenation
Some PICU patients cannot be adequately oxygenated or ventilated using conventional ventilators or HFV. These PICU patients may be candidates for extracorporeal membrane oxygenation (ECMO). The ECMO circuit consists of a venous cannula situated in a large central vein or right atrium, a pump, an oxygenator, an arterial cannula with its tip in the aorta (for venoarterial ECMO) or right atrium (for venovenous ECMO); a bridge that permits flow to bypass the patient; connective tubing; ports for patient and circuit access; and continuous monitors of flow, oxygenation, hemoglobin, and other parameters.
As a rule, ECMO is considered only in patients with a reversible disease causing intractable hypoxemia or severe respiratory acidosis or who require an increase in cardiac output to achieve adequate end organ perfusion. The use of ECMO for severe hypercarbia without severe acidosis, for the prevention of ventilator induced lung injury, or for heart failure as a bridge to a ventricular assist device or heart transplant, is common but is perhaps less well accepted.
ECMO comes with its risks and complications. The ECMO circuit requires systemic anticoagulation, and the most common complication of ECMO remains hemorrhage, which can be from cannulation sites, surgical sites, sites of previous surgical procedures, or in the form of pulmonary and intracerebral hemorrhage. Thromboembolism, infections, and disseminated intravascular coagulopathy are some other commonly encountered problems. Due to the high potential for associated morbidity, the threshold for cannulation in the setting of acute respiratory failure is relatively high. Oxygenation Index (OI) is a valuable measure of mechanical support required to maintain adequate oxygenation, and is measured by:
OI = mean airway pressure ( cm H 2 O ) × FiO 2 ( % ) PaO 2 ( mm Hg )
Recommendations from ECMOnet and Extracorporeal Life Support Organization (ELSO) recommend an OI > 30 or OI > 40, respectively, as indications for initiating ECMO support. If OI is lower than 30 or 40, ECMO may be indicated in the setting of refractory air leak syndromes. While a more extensive discussion of ECMO is beyond the scope of this chapter, suffice it to say, ECMO may be used to provide only gas exchange support (venovenous) or gas exchange and circulatory support (venoarterial). The pediatric pulmonologist should be aware that venovenous ECMO provides only partial gas exchange support and no circulatory support. Thus, oxyhemoglobin saturation values are typically in the 80%–90% range. Venoarterial ECMO can achieve complete cardiorespiratory support and oxyhemoglobin saturations typically are normal, but pulse oximetry may be unreliable, since pulsatile blood flow may be minimal or absent. Bronchoscopy is often required prior to ECMO discontinuation to perform bronchoalveolar lavage and clear mucus plugs that may accumulate while the child is on rest ventilator settings. While anticoagulation certainly increases the risk of bleeding during invasive procedures, successful weaning off ECMO often requires one or more bronchoscopies.
Complications of Mechanical Ventilation
Mucus Plugging
A common problem encountered among mechanically ventilated patients is secretion management. Multiple factors in the ICU place mechanically ventilated children at an increased risk for retained secretions. The inflated endotracheal tube (ETT) cuff causes significant depression of tracheal mucus velocity and disrupts the mucociliary escalator. Furthermore, defects in ciliary function are likely underappreciated and are probably a greater contributor to respiratory failure in the PICU than commonly appreciated (see Chapter 71 , Primary Ciliary Dyskinesia ). The relative immobility and muscular weakness associated with prolonged ICU admissions contributes to weakened cough and pooling of secretions, and depression of spontaneous breathing through the use of sedatives and paralytics compounds the problem by impairing mucociliary clearance and cough reflexes. Fluid restriction and aggressive diuresis also contribute to thickened secretions. Smaller airways in children are especially prone to obstruction by thick, immobile secretions, contributing to ventilation-perfusion (V/Q) mismatch and further worsening of respiratory failure. Acute decompensation can occur if endotracheal tubes or tracheostomy tubes become obstructed with mucus, and suctioning or ETT exchange may be required. Strategies to minimize and treat mucus plugging include humidification, the use of mucolytics, and mechanical disruption of mucus.
Perhaps the simplest and most intuitive medicinal therapy is to ensure adequate humidification of the ventilator circuit. There is clear evidence that drying of the mucosal surface causes mucus adhesion to the epithelium, and heating and humidifying cool medical gases has now become a standard of care during mechanical ventilation. However, the optimum degree of humidification is frequently debated and there are no straightforward or agreed upon methods for monitoring the adequacy of humidification. Secretion volume and consistency, frequency of ETT occlusion, changes in ETT diameter and/or resistance, and suction frequency are often used as surrogate markers for adequate humidification. However, none of these variables are very reliable as they are easily influenced by underlying disease process or concurrent therapies.
Along with standard methods of humidification, nebulization with hypertonic saline (HS, 3% or greater) is frequently used for airway clearance in the setting of thick tenacious secretions that are difficult to suction. Use of hyperosmolar agents such as HS and mannitol was initially studied in patients with cystic fibrosis (CF) and bronchiectasis and showed significant benefit in that population, leading to wider use among mechanically ventilated patients in pediatric ICUs. Theoretically, inhaled HS generates transient osmotic gradients on airway surfaces, drawing water from the submucosal space onto the airway surface. This increased water in the airway lumen reduces the entanglements of the mucin network, thus facilitating mucociliary clearance. Furthermore, in sputum studies, a reduction in the viscoelastic properties, surface tension, and spinability and an increase in the hydration of mucus have been measured in response to HS.
Other adjunctive agents used to treat or prevent mucus plugging include mucolytic agents such as N-acetylcysteine (NAC) and recombinant human deoxyribonuclease (rhDNase). NAC disrupts disulfide bonds that link mucin oligomers and has antioxidant and antiinflammatory properties. NAC was first described as a mucolytic agent in 1963. Several clinical studies were undertaken following the discovery and its use became widespread, particularly among the CF population. Several subsequent trials have failed to show any benefit of using NAC among patients with acute respiratory distress syndrome, ARDS (as well as CF) and a recent meta-analysis of ARDS trials concurred. However, in the setting of mucus hypersecretion and plugging among mechanically ventilated children, its use as a rescue therapy continues due to the lack of clear evidence disproving its efficacy.
DNase is a recombinant endonuclease that cleaves DNA. Its use in the PICU is generally restricted to conditions with purulent respiratory secretions and its use is currently US Food and Drug Administration (FDA) approved in the United States for the treatment of CF lung disease. Multiple studies have evaluated the use of DNase in critical care settings for treatment of atelectasis secondary to mucus plugging in mechanically ventilated patients. Most of these are small underpowered studies, and their findings seem mixed. A recent meta-analysis also recognized the low quality and quantity of existing data, and like NAC, it continues to be used in select patients with thick purulent secretions.
Several mechanical airway clearance techniques are commonly used in the PICU: chest physiotherapy (CPT), intermittent percussive ventilation (IPV), and mechanical insufflation-exsufflation. CPT has been advocated in the field of pediatrics for many years now and is the traditional first-line therapy for secretion management and atelectasis. Several CPT techniques are described and used in mechanically ventilated children: (1) manual percussion with cupping of hand or with a facemask, (2) vibration of the chest wall transmitting energy through the chest wall to loosen or move bronchial secretions, and (3) postural drainage using gravity to move secretions from peripheral airways to the larger bronchi. Despite its historical status and widespread use, evidence for CPT remains weak or absent, and it is not recommended as a routine treatment by the American Association of Respiratory Care.
IPV involves application of high-frequency oscillations to the airways, enhancing secretion clearance when interfaced with conventional mechanical ventilation. The mechanism by which IPV may promote pulmonary secretion clearance is its asymmetrical flow pattern, whereby expiratory flow exceeds inspiratory flow to propel secretions forward. It can be an effective tool in mechanically ventilated patients with refractory atelectasis secondary to mucus plugging. In a small randomized controlled trial, use of IPV in mechanically ventilated pediatric patients produced more clinically important improvement in atelectasis than was observed in children receiving CPT.
Mechanical insufflation-exsufflation, more commonly known as cough-assist, was first introduced in the 1950s, but its use became more widespread in the 1990s. It functions by mechanically inflating the lungs with positive pressure, followed by the rapid application of negative pressure that stimulates a cough. Similar to IPV, cough-assist devices create shear forces in the airway that help mobilize secretions. Typically, positive and negative pressure swings of 30–40 cm H 2 O are applied in repetitions of 3–5 maneuvers to mobilize secretions. This therapy has been used primarily to promote pulmonary hygiene in patients with neuromuscular diseases and is one of the few therapies that are recommended by the American Association of Respiratory Care. It should be used with caution in PICU patients prone to lung derecruitment.
Atelectasis
Atelectasis occurs when airway pressure declines below the critical closing pressure of a given terminal respiratory unit. In a healthy lung, this closing pressure is below atmospheric pressure, but in disease states, it becomes elevated. To reverse atelectasis, airway pressure must be raised higher than the critical opening pressure. This pressure is again elevated in many disease states. Since lung disease in many PICU patients is heterogeneous, maintaining recruitment of one lung region may result in overdistention or injury to another. Mucus plugging may also contribute to atelectasis and should be treated as discussed in the previous section.
The atelectasis present in many PICU patients compromises oxygenation and ventilation secondary to V/Q mismatching. Additionally, pooling of secretions in atelectatic lung segments may also predispose the patient to nosocomial pneumonia. Mucus plugging is probably the commonest cause of atelectasis as discussed above. Other causes of atelectasis include foreign body aspiration, aspiration of secretions or gastric contents, and the regional atelectasis that occurs as a consequence of ARDS.
Nonpulmonary factors often contribute to atelectasis. In the supine patient, abdominal contents push in a cephalad direction, decreasing the functional residual capacity. Complete or partial closure of alveoli in the dependent lung zones results in reduced compliance and increased risk of atelectasis. The most common segment of the lung to develop atelectasis is the left lower lobe, possibly due to compression by the heart in the supine position and its poor drainage. In such cases, atelectasis frequently responds to judicious use of positive end-expiratory pressure (PEEP) that overcomes the closing pressure of smaller airways and thus “stents” them open. PEEP titration studies can be employed at the bedside to determine optimal PEEP that produces maximal lung recruitment and minimizes dead space ventilation. Similarly, recruitment maneuvers can be employed to expand atelectatic lung segments. These maneuvers involve application of elevated airway pressure to increase transpulmonary pressure transiently. This could be through sustained inflation, intermittent sighs, or stepwise increases of PEEP or airway inspiratory pressure. Several studies have demonstrated improvements in oxygenation, lung mechanics, and lung reaeration after application of recruitment maneuvers, but the optimum method of recruitment and the impact on clinical outcome remains under discussion.
The pediatric pulmonologist is commonly consulted in PICU patients with refractory atelectasis due to mucus plugging who may need therapeutic bronchoscopy. Numerous case series have shown fiberoptic bronchoscopy to be moderately effective in recruiting atelectatic lung segments by suctioning secretions under direct visualization. However, it must be recognized that ICU patients might be too unstable from a hemodynamic or respiratory standpoint to undergo bronchoscopy. A significant decrease in PaO 2 and elevation in PaCO 2 , as well as increased auto-PEEP leading to reduced venous return and cardiac output, can be seen during bronchoscopy, and this decline is more pronounced as the duration and amount of suctioning is increased. Hypoxemia eventually returns to baseline but takes up to 15 minutes in patients with healthy lungs and several hours in those patients with parenchymal disease. Studies have also shown elevations in intracranial pressure (ICP) with bronchoscopy despite heavy sedation and paralysis. Decisions regarding bronchoscopy in patients with intracranial pathologies should be made with this information in mind. Importantly, the cause of atelectasis should be established before proceeding with bronchoscopy. While mucus plugging may be amenable to bronchoscopic intervention, atelectasis from other causes (e.g., bronchial compression due to an enlarged cardiac chamber, diaphragmatic dysfunction, and pleural effusion) would not benefit from bronchoscopy. Additionally, while bronchoscopy may temporarily improve atelectasis in patients with severe bronchomalacia or restrictive lung disease, the procedure should be part of a larger respiratory care plan aimed at maintaining lung recruitment. Refer to Chapter 9 , Bronchoscopy and Bronchoalveolar Lavage in Pediatric Patients, for further details.
Ventilator-Induced Lung Injury
Although mechanical ventilation is essential for supporting many patients, it may itself cause lung damage, particularly in disease states such as acute respiratory distress syndrome ARDS, leading to ventilator-induced lung injury (VILI). The development of VILI is triggered by regional overdistension of the alveoli (volutrauma), high airway and alveolar pressures (barotrauma), cyclic opening and collapse of terminal respiratory units (atelectrauma), and inflammatory cell recruitment due to high shear (biotrauma). Barotrauma can manifest as air leaks causing pneumothorax, pneumomediastinum, interlobular emphysema, or subcutaneous emphysema of the face, neck, or chest wall. Barotrauma was the first widely recognized component of VILI and remained unquestioned until the 1980s when Dreyfuss et al. showed that application of high tidal volume and not airway pressure was the major determinant of VILI, introducing the concept of volutrauma. Additionally, heterogeneity of lung compliance in conditions such as ARDS can aggravate the development of VILI as repeated opening and closing of short time constant lung units renders adjacent zones susceptible to shear stresses, creating a vicious cycle of VILI, both at high and low tidal volumes (atelectrauma). A relatively newer concept is that of biotrauma, which suggests that even in the absence of overt tissue injury, such as that caused by barotrauma, unphysiological shear or stress can promote release of proinflammatory cytokines and recruitment of white cells, with resulting lung inflammation.
The application of generous PEEP can be useful in keeping lung segments well recruited and thus avoid atelectrauma. The optimum PEEP level remains unclear and likely varies between individual patients, but several randomized controlled trials and meta-analyses have failed to demonstrate any mortality benefit of using higher levels of PEEP (>12 cm H 2 O). The term “open lung ventilation” is often used to describe the strategy of using higher PEEP to prevent atelectrauma. HFV also offers the advantage of open lung ventilation; continuous and relatively high mean airway pressures keep the alveoli patent at all times as small tidal volumes are administered. As a general rule, pediatric intensivists titrate PEEP or MAP so that at end inspiration the lung is inflated to the approximate volume of the patient’s baseline functional residual capacity.
An understanding of the above mechanisms has led to evidence-based efforts to reduce VILI and produced recommendations for “lung-protective” ventilation strategies, including the use of low tidal volumes and generous PEEP, limiting plateau pressures, and using permissive hypercarbia. The use of lower tidal volumes reduces end-inspiratory stress/strain and theoretically prevents volutrauma. The landmark ARDSnet trial of 2000 compared mechanical ventilation with low tidal volume (6 mL/kg) versus high tidal volume (12 mL/kg) ventilation among adults and successfully established the benefits of lower tidal volume resulting in decreased mortality and an increased number of ventilator-free days. Refer to Chapter 38 , Acute Respiratory Distress Syndrome for further discussion on ARDS and lung protective strategies.
It is important to realize that children in the ICU may have disease processes (pulmonary or otherwise) that prevent optimal ventilation. In such scenarios, escalation of inspiratory pressures would likely improve gas exchange but would also induce barotrauma in the process and prove harmful in the long run. In these situations, it is advisable to limit plateau pressures to 30–35 cm H 2 O and accept higher carbon dioxide levels. While practicing “permissive hypercarbia,” care must be taken to avoid severe respiratory acidosis, particularly in hemodynamically unstable children. Ventilator management in PICU patients commonly involves tradeoffs not necessary outside of the PICU, and strategies are dependent upon careful risk-benefit analysis considered in the context of each patient.
Air Leak Syndromes
Pneumothorax, pneumomediastinum, and subcutaneous emphysema are common air leak syndromes observed in the PICU. One of the commonest causes is aggressive mechanical ventilation and resulting barotrauma or volutrauma with breach of airway integrity. Mechanical ventilation of children with obstructive lung physiology (e.g., asthma, or bronchiolitis obliterans) can also pose challenges with inadequate expiratory flow and resulting hyperinflation, which can cause air leaks. This can occur with both invasive and noninvasive mechanical ventilation. Other causes of air leak include trauma, necrotizing infection, surgical procedure, or endoscopic tracheal and esophageal procedure ( Fig. 37.2 ).
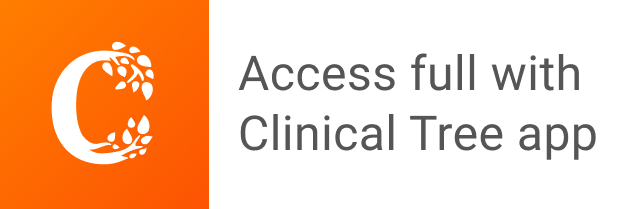