Background
The etiology of flank pain sometimes experienced during the administration of ultrasound contrast agents is unknown. The aim of this study was to investigate whether microbubble ultrasound contrast agents are retained within the renal microcirculation, which could lead to either flow disturbance or local release of vasoactive and pain mediators downstream from complement activation.
Methods
Retention of lipid-shelled microbubbles in the renal microcirculation of mice was assessed by confocal fluorescent microscopy and contrast-enhanced ultrasound imaging with dose-escalating intravenous injection. Studies were performed with size-segregated microbubbles to investigate physical entrapment, after glycocalyx degradation and in wild-type and C3-deficient mice to investigate complement-mediated retention. Urinary bradykinin was measured before and after microbubble administrations. Renal contrast-enhanced ultrasound in human subjects ( n = 13) was performed 7 to 10 min after the completion of lipid microbubble administration.
Results
In both mice and humans, microbubble retention was detected in the renal cortex by persistent contrast-enhanced ultrasound signal enhancement. Microbubble retention in mice was linearly related to dose and occurred almost exclusively in cortical glomerular microvessels. Microbubble retention did not affect microsphere-derived renal blood flow. Microbubble retention was not influenced by glycocalyx degradation or by microbubble size, thereby excluding lodging, but was reduced by 90% ( P < .01) in C3-deficient mice. Urinary bradykinin increased by 65% 5 min after microbubble injection.
Conclusions
Lipid-shelled microbubbles are retained in the renal cortex because of complement-mediated interactions with glomerular microvascular endothelium. Microbubble retention does not adversely affect renal perfusion but does generate complement-related intermediates that are known to mediate nociception and could be responsible for flank pain.
Contrast-enhanced ultrasound (CEU) imaging, including myocardial contrast echocardiography, relies on the acoustic detection of gas-filled microbubble contrast agents. Microbubbles that are approved for clinical use are encapsulated by albumin or lipid surfactants and behave similarly to erythrocytes in the microcirculation of the heart and skeletal muscle. Although the safety of commercially produced microbubbles has been established by trials that evaluated serious reactions, postmarketing surveys and retrospective studies have revealed that flank pain is one of the most frequent nonserious adverse reactions that can necessitate termination of lipid microbubble administration.
Although the etiology of flank pain with ultrasound contrast agents is unknown, similar symptoms have been reported with high doses of liposomal drug preparations. It has been proposed that liposome-related flank pain is caused by complement (C′) activation, which occurs in proportion to total lipid dose. Lipid microbubbles can also promote C′ activation, resulting in deposition of human C3b on the microbubble surface, particularly when highly charged lipids are present in the shell. Complement proteins on the shell surface can mediate microbubble adhesion to activated leukocytes and vascular endothelium. Hence, it is possible that C′ may mediate renovascular retention of microbubbles and produce flank discomfort through either physical obstruction to flow or local production of vasoconstrictors and/or nociceptive peptides produced downstream from C′ activation.
Our aims were to evaluate whether microbubble retention occurs within the renal microcirculation in mice and humans and to determine the histologic location of retention by confocal microscopy. To evaluate the potential mechanisms of retention, we assessed for size-dependent lodging and evaluated the effects of microbubble charge, competence of the C′ system, and integrity of the glycocalyx, which influences cell-microbubble interaction.
Methods
Microbubbles for Murine Experiments
Lipid-stabilized decafluorobutane microbubbles were prepared by sonication of a gas-saturated aqueous dispersion of lipids. Microbubbles with a near neutral charge (MB) were prepared with a dispersion of 2.0 mg/mL distearoyl phosphatidylcholine and 0.5 mg/mL polyoxyethylene-40-stearate. Microbubbles with a net negative charge (MB − ) and microbubbles with a net positive charge (MB + ) were prepared by adding either distearoyl phospho-L-serine and distearoyl trimethylammonium propane (0.1 mg/mL), respectively. Microbubble ζ potential was determined by laser Doppler velocimetry (ZetaPlus; Brookhaven Instruments, Holtsville, NY) in 1 mmol/L potassium chloride. The results of three separate measurements were averaged. Separation of microbubbles into small size population was performed by flotation-centrifugation at 400 g for 1 min and isolation of the turbid subnatant. For confocal microscopy, lipid microbubble shells were fluorescently labeled by adding dioctadecylindocarbocyanine to the suspension before sonication. Studies with albumin-shelled octafluoropropane microbubbles were performed with Optison (GE Healthcare, Princeton, NJ). Microbubble concentration and size distribution were determined by electrozone sensing (Multisizer III; Beckman Coulter, Fullerton, CA).
Animal Preparation
The study was approved by the Animal Care and Use Committee of the Oregon Health & Science University. Male wild-type C57Bl/6 mice and C3-deficient (C3-/-) mice (Jackson Laboratory, Bar Harbor, ME) were studied at 8 to 12 weeks of age. Mice were anesthetized with inhaled isoflurane (1.0%–1.5%). Body temperature was maintained at 37°C with a heating platform. A jugular vein was cannulated for the administration of microbubbles.
CEU in Mice
Renal CEU was performed from a dorsal approach with a linear-array probe (Sequoia; Siemens Medical Systems USA, Inc, Mountain View, CA) using phase-inversion amplitude-modulation imaging at 7 MHz and a mechanical index (MI) of 0.16. Microbubbles were injected as an intravenous bolus injection. Imaging for retained microbubbles was performed 8 min after injection. After initial image acquisition, several high-power (MI = 1.0) frames were applied to destroy microbubbles in the sector. Signal from retained microbubbles was calculated by digitally subtracting several averaged frames obtained >5 sec after the destructive pulse sequence from averaged frames obtained before the destructive pulse sequence. Intensity was measured from a region of interest placed on the entire kidney guided by fundamental two-dimensional imaging acquired after each CEU imaging sequence.
Murine CEU Protocols to Assess Retention
Kidney retention of lipid microbubbles was evaluated with bilateral renal CEU using the following five conditions: (1) injection of MB at escalating doses from 1 × 10 4 to 1 × 10 6 ( n = 12); (2) injection of 1 × 10 6 MB with either normal size dispersion or size separated to obtain the small MB population ( n = 5); (3) injection of 1 × 10 6 MB in C3-/- mice ( n = 5); (4) injection of 1 × 10 6 MB, MB + , and MB − performed in random order ( n = 5); and (5) injection of 1 × 10 6 MB, MB + , and MB − in random order before and after degradation of the glomerular glycocalyx produced by intravenous administration of hyaluronidase (1.5 × 10 4 U/kg, 60 min prior) and heparinase III (8.2 U/kg, 15 min prior) ( n = 5). To evaluate relative retention of albumin and lipid microbubbles, CEU was performed in three mice after the injection of MB (5 × 10 5 ) and albumin-shelled microbubbles at medium and high doses (5 × 10 5 and 1 × 10 6 ).
Confocal Microscopy for Microbubble Retention
In five wild-type mice, 1 × 10 6 dioctadecylindocarbocyanine-labeled MB were injected intravenously. After 8 min, 100 μg fluorescein isothiocyanate–labeled Lycopersicon esculentum lectin was injected to label the endothelium. Mice were euthanized, and the blood pool was flushed by isothermic saline (5 mL) infused via the left ventricle at 80 to 100 mm Hg. Confocal fluorescent microscopy (FW1000; Olympus, Center Valley, PA) was performed using nonfixed thick sections (1 mm) of the kidney.
Renal Blood Flow
Changes in renal microvascular blood flow produced by microbubble injection in mice was evaluated using fluorescent microscopheres ( n = 3). An arterial cannula was placed in the carotid artery and advanced retrograde into the aorta. Fluorescently labeled microspheres (5 × 10 4 ) with a diameter of 15 μm (Dye Track-F; Triton Technology, San Diego, CA) were suspended in 20 μL saline and injected over 1 min. A second injection of microspheres labeled with a different fluorophore was repeated 8 min after intravenous injection of 1 × 10 6 MB. Both kidneys were removed, cut into 1-mm-thick sections, and the number of microspheres labeled with each fluorophore was quantified by fluorescent microscopy.
Urinary Bradykinin
Urine was sampled through a urethral microcatheter at baseline and 5 to 10 min after intravenous injection of 1 × 10 6 MB. Bradykinin concentration was measured by enzyme-linked immunosorbent assay (Phoenix Pharmaceuticals, Burlingame, CA).
Histology
Renal tissue was obtained 24 hours after the intravenous injection of 1 × 10 6 MB, fixed, and stained with hematoxylin to evaluate for inflammatory cell infiltration ( n = 3). Complement regulatory protein expression in the glomeruli on dual fluorescent confocal microscopy was assessed ( n = 2) by intravenous injection of 5 μg/g phycoerythrin-labeled monoclonal antibody against murine decay-accelerating factor (CD55) (RIKO3; Biolegend, San Diego, CA) followed 30 min later by 100 μg fluorescein isothiocyanate–labeled lectin.
Human CEU Imaging
The study was approved by the human investigational review board at Oregon Health & Science University. Fourteen hemodynamically stable outpatients who were undergoing contrast echocardiography for endocardial delineation were recruited. Exclusion criteria included severe left ventricular systolic dysfunction, heart failure, renal insufficiency, active inflammatory or autoimmune disorder, or immunomodulatory therapy. Renal CEU was performed with a phased-array transducer (iE33; Philips Medical Systems, Andover, MA) using amplitude-modulation imaging at 1.6 MHz and an MI of 0.12 to 0.14. Images were acquired 7 to 10 min after the completion of an infusion of lipid-shelled octafluoropropane microbubbles (Definity; Lantheus Medical Imaging, North Billerica, MA) as part of their clinically indicated contrast echocardiographic studies (dose range, 0.8–1.2 mL). Images were acquired before and after several high-power (MI = 1.0–1.1) frames, and image analysis was performed similarly to murine experiments.
Statistical Analysis
Data are expressed as mean ± SD unless otherwise mentioned. Normally distributed continuous variables were analyzed using unpaired Student’s t tests (two sided). Paired t tests were used for microbubble retention data before and after glycocalyx disruption and for microsphere blood flow data. One-way analysis of variance was used to compare the effects of microbubble charge, and when significant, post hoc analysis with unpaired Student’s t tests and Bonferroni correction for multiple comparisons was performed. Urinary bradykinin levels were compared using Wilcoxon’s test. The dose-intensity relationship was evaluated using linear regression analysis.
Results
Retention of Microbubbles in the Renal Microcirculation
In wild-type mice, signal enhancement on CEU from retained lipid MB was consistently detected 8 min after their intravenous injection at a time when most microbubbles had cleared from the circulating blood pool. Signal enhancement from retained MB was linearly related to dose ( Figure 1 A). Signal was proportionally much greater for lipid microbubbles than for albumin microbubbles ( Figure 1 B). Signal enhancement for MB was observed primarily in the region of the renal cortex ( Figure 1 C). Confocal microscopy indicated that this signal was attributable primarily to MB retention within the cortical glomerular microvessels ( Figure 1 D). There was no evidence for extravascular transit of MB. Retention of MB in peritubular vessels was uncommon.

Mechanisms for Microbubble Retention
To investigate physical entrapment as a mechanism for MB retention, renal CEU was performed with MB preparations either with conventional size distribution or after size segregation to eliminate large MB. Size separation produced a modest reduction in the mean microbubble diameter, but the proportion of microbubbles > 5 mm, which represents the minimal glomerular capillary diameter, was reduced by >85% ( Table 1 ). In vitro CEU imaging experiments using dose escalation in a water bath indicated a similar intensity for the conventional and size-segregated agent, thereby eliminating the need for dose adjustments for size-segregated MB to compensate for any reduction in microbubble signal in the in vivo studies ( Figure 2 ). Signal enhancement from retained microbubbles in the kidney was minimally reduced when using the small size-segregated population ( Table 1 ), arguing against size-dependent lodging as a major mechanism.
Variable | Conventional | Size segregated (small population) |
---|---|---|
Microbubble diameter (μm) | 2.2 ± 1.5 | 1.7 ± 0.7 |
Diameter > 5 μm | 3.82% | 0.44% |
Renal signal intensity (video intensity units) ∗ | 25 ± 3 | 21 ± 2 |
For experiments investigating the effect of net microbubble surface charge, ζ potential was −6 mV for MB, −38 mV for MB − , and +47 mV for MB + . Altering the charge in either an anionic or cationic direction resulted in mild differences in microbubble retention under basal conditions ( P = .03, ANOVA), with slightly greater retention for MB + ( Figure 3 A). However, differences did not meet statistical significance after correcting for multiple comparisons. Signal for all three agents was almost identical after systemic treatment with hyaluronidase and heparinase to degrade the glycocalyx, arguing against any interactions with the glomerular glycocalyx. Renal retention of MB was reduced by almost 90% in C3-deficient compared with wild-type mice ( Figure 3 B). Confocal microscopy demonstrated that CD55, which is an endothelial-associated complement regulatory protein, was highly expressed on the glomerular capillaries ( Figure 3 C). Urinary bradykinin, which was used as a marker for glomerular complement activation that is also involved in nociception, was significantly increased after microbubble injection ( Figure 4 A).
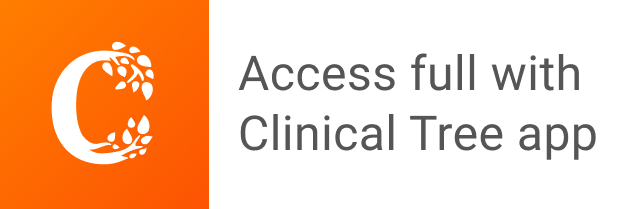