Background
When activated by the sympathetic nervous system, brown adipose tissue (BAT) increases energy expenditure to produce heat. Augmenting BAT mass or increasing BAT activation could potentially be used to decrease obesity. Noninvasive methods to detect and monitor BAT mass are needed. Contrast ultrasound can estimate BAT blood flow and is able to measure the perfused volume of an organ and thus its mass. The objective of this study was to evaluate whether contrast ultrasound could characterize BAT mass in two mouse models of obesity: wild-type mice fed a high-fat diet and mutant db / db mice.
Methods
Contrast ultrasound of BAT (Definity 2 μL/min; 14-MHz linear probe) was performed before and after stimulation of BAT with norepinephrine (NE). BAT replenishment curves were obtained, and blood flow was estimated by the product of the curve’s plateau and slope. Additionally, consecutive two-dimensional images of perfused BAT were acquired at 1-mm intervals after stimulation with NE and used to assess BAT volume and mass.
Results
BAT blood flow increased after NE infusion in all mice studied. Blood flow response to NE was similar in wild-type mice fed either a low-fat diet or a high-fat diet. BAT blood flow was lower in db / db mice than in wild-type mice ( P = .02). Contrast ultrasound–derived BAT mass was correlated with BAT mass obtained at necropsy ( R 2 = 0.83, P < .001). BAT mass was higher in mice fed a high-fat diet than in those fed a low-fat diet.
Conclusions
Contrast ultrasound can be used to estimate BAT mass in mice when BAT vascularization is not significantly impaired. This noninvasive technique may potentially allow the serial evaluation of therapies designed to augment BAT mass.
Obesity affects >500 million adults and is accompanied by increased morbidity and mortality. Weight gain results from an imbalance between energy intake and expenditure. As efforts to decrease energy intake by diet or other treatments are met with limited weight loss and potentially large side effects, increasing energy expenditure has become an attractive potential therapeutic target for weight loss.
When activated by the sympathetic nervous system, brown adipose tissue (BAT) increases energy expenditure by using fatty acids and glucose to produce heat. The thermogenic process is mediated by the mitochondrial uncoupling protein 1 (UCP1), which allows protons produced by lipolysis and glycolysis to reenter the mitochondrial matrix, while generating heat instead of adenosine triphosphate.
The presence of BAT has been reported for decades in rodents (mainly between the scapulae and in smaller depots in the cervical, perivascular, and perirenal regions ) and human infants. The interest in BAT physiology, however, considerably increased after recent reports demonstrated the presence of functional BAT detected by 18 F-fluorodeoxyglucose (FDG) positron emission tomography (PET) in adult humans. Although the clinical role of BAT has not been thoroughly elucidated, it has been calculated that 50 g of maximally activated BAT could consume 20% of an adult human’s daily energy intake ; extensive research is under way to develop therapies that increase the mass and/or activity of BAT as a potential weight loss therapy.
One obstacle in research targeted toward BAT is the lack of noninvasive in vivo technique to delineate its mass. Such a technique would allow serial measurements of BAT mass, useful in particular in the investigation of potential BAT-based therapies. Activation of BAT is associated with a marked increase in its local blood flow. Taking advantage of the dense vascularization of BAT, we recently validated contrast ultrasound (CU) as a novel method to estimate BAT blood flow in vivo in mice.
The present study relied on the fact that CU may be used to measure the perfused volume of an organ, which is correlated with its mass. Our first objective was to investigate whether CU could be used to assess BAT mass in mice.
Interestingly, in humans, the response of BAT to stimulation by cold (assessed by FDG PET) is inversely correlated with body mass index in older adults, suggesting that obese older adults may have impaired BAT activation or decreased BAT mass. This relationship does not seem to be present in younger adults.
The models in mice attempt to reproduce some of the features of human obesity. High-fat diet (HFD) feeding represents a model of environmental modifications and is associated with mild obesity. In contrast, db / db mice have a genetic mutation that inactivates the leptin receptor and develop severe obesity and diabetes. In the present study, the BAT in these two obesity models was characterized using ultrasound. It was anticipated that both models would allow investigation of the ability of CU to characterize the mass and function of BAT, as these phenotypically different conditions may be associated with distinct BAT mass and functionality.
Methods
All animal studies were approved by the Subcommittee on Research Animal Care of the Massachusetts General Hospital (Boston, MA). Four-month-old to 5-month-old male C57BL/6J (wild-type [WT]) and C57BKS.Cg- Dock7 m +/+ Lepr db /J ( db / db ) mice obtained from Jackson Laboratory (Bar Harbor, ME) were studied. The db / db mice ( n = 6) and WT mice ( n = 6) were fed a standard diet (11.8% kcal from fat; Prolab Isopro RMH 3000 5P75, LabDiet, Richmond, IN). In a separate experiment, male WT mice were fed either a low-fat diet (LFD; n = 7) (10% kcal from fat; D12450B, Research Diets, New Brunswick, NJ) or an HFD ( n = 8) (60% kcal from fat; D12492, Research Diets). Both the LFD and the HFD were started at 2 months of age and maintained for 2 months. Five mice of each group were used for blood flow measurements, but all mice were used for mass measurement. It has been reported that gender does have an effect on BAT function (female gender appears to be accompanied by an increase in BAT function), for reasons that have not yet been totally elucidated. Therefore, only male mice were studied, so as not to increase the variability of the experiments.
CU
Mice were weighed and anesthetized with an intraperitoneal injection of ketamine (100 mg/kg) and xylazine (10 mg/kg). Tracheal intubation was performed, and volume-controlled ventilation was initiated at a respiratory rate of 110 to 120 breaths/min and a tidal volume of 10 μL/g. For the assessment of db / db mice, the right jugular vein was catheterized using a fluid-filled PE-10 catheter. A less invasive approach using tail vein catheterization (with a 31-gauge needle) and lower concentrations of ketamine (80 mg/kg) and xylazine (8 mg/kg) was used for the study of HFD-fed mice.
Mice were placed in the prone position. Body core temperature was monitored and kept constant at 37°C (DC Temperature Control System; FHC, Bowdoin, ME). Perflutren lipid microbubbles (Definity; Lantheus Medical Imaging, Inc., North Billerica, MA) were diluted (1:10 in sterile 0.9% saline solution) and continuously infused intravenously at a rate of 20 μL/min into the right jugular vein or the tail vein.
Estimation of BAT Mass by CU
Acquisition
Mice were placed on a mobile platform while a 14-MHz ultrasound linear transducer (Acuson Sequoia C512; Siemens Medical Solutions USA, Inc., Mountain View, CA) was maintained in a fixed position above the interscapular area using a clamp ( Figure 1 A). Interscapular BAT was identified by localization of anatomic landmarks (scapulae, Sulzer’s vein). To better delineate the perfused volume of BAT, norepinephrine (NE; Bedford Laboratories, Bedford, OH) was infused intravenously at 1 μg/kg/min to stimulate BAT. Microbubbles were infused simultaneously. BAT was scanned (CPS mode; frame rate, 30 Hz; mechanical index, 0.24) by moving the platform in 1-mm intervals parallel to the long axis of the mouse spine. Scanning of BAT was performed while the microbubbles were infused continuously. Each view was acquired for 1 sec and stored immediately. The transducer was then moved and the next view acquired.

Analysis
The perimeter of each BAT lobe was traced, and the area (in square millimeters) was measured for each platform position (9–14 positions for each lobe; Figures 1 B and 1C). The volume (in cubic millimeters) of each lobe was calculated using Simpson’s rule. The mass was then calculated by multiplying the volume by the specific density of BAT (0.98).
Estimation of BAT Blood Flow by CU
Acquisition
CU was performed before and 10 min after the start of a continuous infusion of 1 μg/kg/min NE. For each acquisition, images centered on the BAT were acquired with the same settings as described above, before and for 10 sec after a burst of 10 high-energy frames (mechanical index, 1.8). The right kidney and the right quadriceps femoris muscle were also imaged. Two sets of images were obtained, both at baseline and after NE stimulation.
Analysis
Regions of interest were manually positioned in the BAT, kidney cortex, and quadriceps femoris muscle, as previously described. The average signal intensity within the region of interest was automatically measured for each frame (syngo ACQ; Siemens Medical Solutions USA, Inc.). The relationship of the signal intensity over time after bubble destruction was obtained in each region of interest and fitted to an exponential function: y = A × (1 − e −β t ), where y is the signal intensity, A is the plateau intensity, and β is the initial slope of the replenishment curve. Blood flow was estimated by calculating the product of A and β.
Histology and Immunohistochemistry
After CU was completed, mice were euthanized, and the BAT was carefully dissected and weighed. BAT resection was performed using a dissecting microscope. BAT was distinguished from surrounding muscles and white adipose tissue on the basis of the color and the consistency of the tissues. BAT was embedded in paraffin and 5-μm-thick slides were obtained at 1-mm intervals and stained using hematoxylin and eosin.
UCP1 expression in BAT was localized by immunohistochemistry using rabbit anti-UCP1 antibody (1:100, ab10983; Abcam, Cambridge, MA) and goat antirabbit immunoglobulin G antibody (1:200, Sigma 41176; Sigma-Aldrich, St. Louis, MO). Samples were then incubated in a working solution of horseradish peroxidase conjugate (250 μL), and 0.03% diaminobenzidine (250 μL) was applied to the surface of the slide. Samples were counterstained in hematoxylin, dehydrated with sequential ethanol and xylene immersion, and mounted with DPX mountant for histology (Sigma-Aldrich).
Capillary density was measured by incubating BAT sections of WT and db / db mice with biotinylated Griffonia simplicifolia lectin I and staining them with the Vectastain ABC immunoperoxidase system (Vector Laboratories, Burlingame, CA). The number of capillaries was then counted per square millimeter. Five fields were analyzed for each mouse ( n = 3 mice in each group) at a magnification of 400×.
Statistical Analysis
Statistical analysis of the results was performed using the JMP statistical software package (SAS Institute Inc., Cary, NC). All values are expressed as mean ± SEM.
Ultrasound-estimated BAT mass was correlated with the BAT mass measured at necropsy using simple regression. Ultrasound-estimated BAT mass was compared in LFD-fed and HFD-fed mice using an unpaired Student’s t test. For comparison of the response of BAT blood flow to NE in LFD-fed and HFD-fed WT mice and in db / db and WT mice, the results were analyzed using two-way analysis of variance for repeated measurements. If the interaction of NE and diet or strain was significant, unpaired Student’s t tests were used to compare ultrasound parameters between groups at the same time point. P values < .05 were considered significant.
The interobserver reliability of CU-estimated mass measurements was performed by two independent observers (M.C. and L.E.) in seven randomly chosen mice (four mice in the HFD group and three mice in the LFD group). To measure intraobserver reliability, a single observer (L.E.) repeated the measurements on the same loops several weeks after the first measurement set. Intraobserver and interobserver variability was calculated as the difference between the two observations divided by the means of the observations and expressed as both absolute numbers and percentages. To estimate the variability between the curves obtained in each mouse, a pooled standard deviation for each of the measurements is also reported.
Results
Intraobserver and Interobserver Variability
The intraobserver variability for CU-estimated mass measurements as assessed by the mean ± SD of the error was 19.1 ± 19.9 mg (10.0 ± 8.9%) ( Figure 2 A). The interobserver variability for CU-estimated mass measurements was 17.9 ± 22.2 mg (10.8 ± 10.7%) ( Figure 2 B).
Validation of CU-Derived BAT Mass
The CU-derived BAT mass was compared with the mass obtained at necropsy in LFD-fed and HFD-fed mice. A close correlation was found between BAT mass derived from CU and that measured at necropsy (CU-derived BAT mass = 1.3 × BAT mass at necropsy − 104, R 2 = 0.83, P < .001; Figure 3 A). BAT mass at necropsy was overestimated by CU; this effect was maximal for the lowest mass values ( Figure 3 B).
Effect of Obesity on Body Weight and BAT Mass
The body weight of WT mice fed an HFD for 2 months was greater than that of WT mice fed an LFD (40.8 ± 2.5 vs 30.6 ± 0.9 g, P = .003). CU-estimated BAT mass was 213 ± 19 mg in HFD-fed mice and 164 ± 9 mg in LFD-fed mice ( P = .03; Figure 4 ).
The body weight of db / db mice was markedly greater than that of WT mice (56 ± 2 vs 30 ± 1 g, P < .001). The two BAT lobes could be visualized in the db / db mice ( Figure 5 ), but BAT blood flow was too low to precisely measure BAT mass using CU.
Effect of HFD Feeding on BAT, Kidney, and Muscle Blood Flow
Before infusion of NE, estimated BAT blood flow was low in LFD-fed mice. Estimated BAT blood flow increased markedly after stimulation of BAT with NE ( P = .005; Table 1 ). Similarly, the estimate of BAT blood flow was low at baseline in HFD-fed mice and increased after NE infusion ( P = .003). There was no difference in the NE-induced increase in the estimate of BAT blood flow between LFD-fed and HFD-fed mice. Estimated kidney and muscle blood flow was similar in LFD-fed and HFD-fed mice at baseline and did not change during NE infusion.
Variable | LFD ( n = 5) | HFD ( n = 5) | ||
---|---|---|---|---|
Baseline | NE infusion at 1 μg/kg/min | Baseline | NE infusion at 1 μg/kg/min | |
BAT (dB/sec) | 0.5 ± 0.1 | 12.8 ± 2.2 ∗ | 0.9 ± 0.2 | 11.2 ± 1.7 ∗ |
Kidney (dB/sec) | 9.1 ± 1.2 | 8.0 ± 1.7 | 10.4 ± 0.7 | 9.1 ± 1.8 |
Muscle (dB/sec) | 0.5 ± 0.2 | 0.2 ± 0.03 | 0.5 ± 0.2 | 0.3 ± 0.1 |
BAT, Kidney, and Muscle Blood Flow in db / db Mice
In WT mice fed a standard diet, the estimated BAT blood flow was low before NE infusion and increased 13-fold after stimulation with NE ( P = .01; Table 2 ). The estimate of BAT blood flow in db / db mice was lower than that of WT mice before NE ( P = .035) and increased after stimulation with NE ( P = .04). The estimated blood flow in BAT after infusion of NE was markedly lower in db / db mice than in WT mice ( P = .02). Estimated kidney blood flow was similar at baseline and decreased in both groups after NE infusion ( P = .048 in WT mice and P = .049 in db / db mice). The estimate of muscle blood flow was low at baseline in both WT and db / db mice and remained unchanged after stimulation with NE.
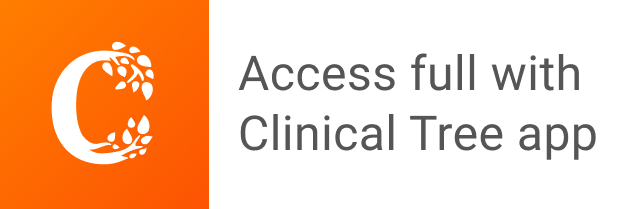