Chapter 8 Regenerative Medicine
Stem Cell Sources
Stem cells are defined by their capacity to self-renew and differentiate into multiple functional cell types (Table 8-1). Traditionally, they have been divided into two main groups based on their potential to differentiate (Fig. 8-1). Pluripotent stem cells (embryonic) can differentiate into every cell of the body, whereas multipotent stem cells (adult) can differentiate into multiple, but not all, cell lineages. In addition to the traditional stem cell classification, a new class of stem cells has recently been described—induced pluripotent stem (iPS) cells—which are derived from genetically reprogrammed adult cells. These diverse cell populations hold much promise to provide researchers and clinicians with an expanded armamentarium to treat diseased and dysfunctional organs.
Table 8-1 Definitions of Stem Cell-Related Terms
TERM | DEFINITION |
---|---|
Totipotent | Ability to form all cell types and lineages of organism (e.g., fertilized egg) |
Pluripotent | Ability to form all lineages of the body (e.g., embryonic stem cells) |
Multipotent | Ability of adult stem cells to form multiple cell types of one lineage (e.g., mesenchymal stem cells) |
Unipotent | Cells form one cell type (e.g., follicular bulge skin stem cells) |
Reprogramming | Dedifferentiation into an embryonic state; can be induced by nuclear transfer, genetic manipulation, viral transduction, and related methods |
Embryonic Stem Cells
During development, two distinct lineages emerge during the transition from morula to blastocyst, the trophoectoderm and the inner cell mass. Embryonic stem cells (ESCs) are immortal cell lines derived from the inner cell mass of the blastocyst. The two hallmark characteristics of ESCs are their unlimited in vitro self-renewal capacity and their ability to differentiate into all somatic cell types.1 A number of transcription factors, most prominently Oct4, Sox2, and Nanog, are essential regulators that ensure the maintenance of pluripotency while suppressing differentiation.2 The two glycolipid antigens SSEA3 and SSEA4 are operational cell surface markers used to identify human ESCs.3 Since the successful isolation of mouse and human ESCs, their potential for cell replacement therapy and regenerative medicine has been widely acknowledged.4 Both mouse and human ESCs have demonstrated an in vitro capacity to form cardiomyocytes, hematopoietic progenitors, neurons, skeletal myocytes, adipocytes, osteocytes, chondrocytes, and pancreatic islet cells when cultured under specific growth factor conditions.5,6
However, a number of limitations currently exist regarding the use of human ESCs in regenerative medicine. Although pluripotentiality and unlimited ability for self-renewal make ESCs attractive for cell replacement therapy, these same characteristics simultaneously translate into unregulated differentiation and formation of teratomas and teratocarcinomas. These tumors contain differentiated cells that contain all three primary germ layers, as well as undifferentiated pluripotent stem cells. This tendency to form tumors has been observed when ESCs are transplanted into mice, raising the concern that human ESC–based therapy may also lead to unwanted tumor formation.1 Without the elimination of this possibility, the clinical use of ESC-derived tissue will remain limited.
In addition, any cell-based therapy must be free of animal contaminants that might contain pathogens or elicit an immune reaction after transfer to a host. Both mouse cell and human ESC lines are generally grown on a mouse-derived feeder layer of fibroblasts that provides additional factors that promote ESC proliferation as well as inhibit their differentiation. One example of possible animal product contamination is the demonstration that human ESCs grown on mouse feeder cells express a nonhuman sialic acid that could elicit a host’s immune response.7 Concerns have also been raised over the possible transfer of murine viruses from feeder layers to human ESCs. Many laboratories are working to solve this problem, with some studies demonstrating the ability to culture human ESCs under serum-free defined medium conditions on human cell-derived feeders or under feeder-free conditions.8
Somatic Cell Nuclear Transfer
Somatic cell nuclear transfer (SCNT), also referred to as reproductive cloning, involves the transfer of nuclei from postnatal somatic cells into an enucleated ovum. Mitotic divisions of this cell in culture lead to the generation of a blastocyst capable of yielding a whole new organism. Major advances in this field came in 1997 with the production of a normal sheep (Dolly),9 and this procedure has been reproduced in other mammals, including mice, cattle, pigs, cats, and dogs.10
These experimental studies suggest that a similar approach using SCNT might work in humans for therapeutic cloning, whereby human ESCs produced by this approach could be subsequently differentiated into therapeutically useful cells and transplanted back into patients with degenerative diseases. A recent report on primate ESC lines, which were derived from rhesus macaque SCNT blastocysts using adult male skin fibroblasts as nuclear donors, is an important step in this direction.11
However, similar to human ESCs, SCNT is embroiled in an ethically complex debate about the moral status of created embryo and concerns about obtaining human unfertilized eggs. The technical limitations of this procedure have also dampened early enthusiasm, because several studies have reported less than 10% efficiency in the derivation of SCNT-generated ESCs.12 Despite the controversy, SCNT and therapeutic cloning may still be a promising means to generate genetically matched stem cell lines. Long-lasting cell lines from patients with diseases created via SCNT can be used to screen potentially useful drugs or other treatments and may provide replacement cells for damaged organs.
Induced Pluripotent Stem Cells
Given the complex logistical and ethical considerations surrounding donated oocytes for SCNT, alternatives that recapitulate the reprogramming process in vitro while avoiding the need for oocytes altogether are ultimately preferable. A groundbreaking study in 2006 by Takahashi and Yamanaka13 defined a specific set of transcription factors, Oct4, Sox2, Klf4, and cMyc, that were sufficient to reprogram adult mouse fibroblasts back into a pluripotent state, thus creating ESC-like induced pluripotent stem (iPS) cells. Takahashi and coworkers14 quickly demonstrated that the same combination of transcription factors is sufficient for the pluripotent induction of human cells as well. The ease and reproducibility of generating iPS cells compared with SCNT has raised the hope that iPS cells might fulfill much of the promise of human ESCs in regenerative medicine.
It is widely accepted that mouse and human iPS cells closely resemble molecular and developmental features of blastocyst-derived ESCs.13,15 A number of research groups have shown that iPS cells injected into immunodeficient mice give rise to teratomas comprising all three embryonic germ layers, similar to ESCs. In addition, when injected into blastocysts, iPS cells generated viable high-contribution chimeras (mice that show major tissue contributions of the injected iPS cells in the host mouse) and contributed to the germline.13,15 Furthermore, using reverse transcription polymerase chain reaction (RT-PCR) assays and immunocytochemistry, studies have shown that iPS cells express key markers of ESCs.
However, recent evidence has demonstrated that iPS cells are not identical to ESCs. Global gene expression analysis comparing iPS cells with human ESCs using microarrays has demonstrated that approximately 4% of the over 32,000 analyzed genes had more than a fivefold difference in expression.16 Furthermore, chimeras and progeny mice derived from iPS cells had higher than normal rates of tumor formation than those derived from ESCs, which in some cases may have been caused by reactivation of the transfected c-Myc oncogene.17 These key differences need to be elucidated further to define the safety of iPS cell use in regenerative medicine.
Another potential complication with the generation of iPS cells is the use of retroviral and lentiviral vectors to activate the necessary reprogramming transcription factors. Specifically, the viral genome could be inserted near endogenous genes, resulting in gene activation or silencing. This risk of insertional mutagenesis could lead to uncontrolled modification of the genome, with potential development of cancer. Much progress has been made in generating integration-free murine iPS cells, and various recent studies using adenoviral, plasmid-based, and recombinant protein-based strategies have reported that viral integration is not required for the reprogramming process.18,19 Even without viral integration, the safety of iPS cells needs to be rigorously tested, because all essential reprogramming factors are oncogenes and their overexpression has been linked with cancers.20 The characterization of iPS cells will be enhanced by ongoing improvements in the high-resolution analysis of genomic integrity via DNA sequencing technology to identify even minor deletions, inversions, or loss of individual alleles readily.
The generation of iPS cells is likely to create a major impact on regenerative medicine. These iPS cells can be generated from human adipose-derived stem cells (ASCs) in a feeder-free condition with a faster speed and higher efficiency than comparable strategies targeting adult human fibroblasts.21 Given the ease of isolating a large quantity of ASCs from lipoaspirates, ASCs could be an ideal autologous source of cells for generating individual-specific iPS cells.
The therapeutic potential of iPS cells has been demonstrated in several preclinical models. For example, Wernig and colleagues have demonstrated that neurons derived from reprogrammed fibroblasts could alleviate the disease phenotype in a rat model of Parkinson’s disease.22 Using a humanized sickle cell anemia mouse model, Hanna and associates23 have shown that the genetic defect could be corrected using transplantation of hematopoietic stem cells (HSCs) derived from iPS cells (derived from fibroblasts of those mice) that had homologous recombination of an intact wild-type β-globin gene. Although these early preclinical studies are very promising, iPS cell technology will require further refinement before clinical applications can be feasible.
Fetal Stem Cells
Although less prominently discussed, fetal stem cells represent another source for a regenerative building block with clinical potential. Fetal stem cells can be derived from fetal blood, liver, bone marrow, amniotic fluid, and placenta, and are rich in a population of stem cells that proliferate more rapidly and exhibit greater multipotentiality than adult stem cells.24,25 Fetal stem cells have been found to expand in culture for at least 20 passages, and their capacity for adipogenic, osteogenic, and chondrogenic differentiation has been demonstrated under appropriate culture conditions.26 In addition, transplantation into a xenogeneic sheep model has shown the ability of these cells to engraft and undergo site-specific tissue differentiation.
Adult Stem Cells
Once embryonic development has completed, humans and other complex organisms lose their cache of embryonic stem cells. During adult life, the regenerative capacity of tissues and organs is maintained by adult stem cells, which reside in mature tissues and in general repositories throughout bone marrow and adipose tissue. Unlike embryonic stem cells and induced pluripotent stem cells, adult stem cells are multipotent; they can differentiate into some but not all tissue lineages and are typically confined to a certain tissue type and microenvironment, usually termed a stem cell niche.27 The most studied and best characterized adult stem cell types is the hematopoietic stem cell, which has served as the experimental paradigm for basic studies into the biology of adult stem cell biology.28 Recently, much insight has been gained into the organization and function of mesenchymal stem cells and adipose stromal cells, which have shown considerable promise for the field of regenerative medicine.
Tissue-Specific Stem Cells
Given the frequent cellular turnover and significant regenerative capacity of epithelial organs such as the cornea, small intestine, and skin,29 it is not surprising that these tissues harbor robust resident stem cell populations. However, resident stem cells have also been isolated from organ systems that were thought to have little or no regenerative capacity, such as cardiac tissue30 and neural tissue,31 suggesting that most or all mature mammalian tissues and organs have corresponding stem cell populations that play some role in local tissue homeostasis and organ regeneration. These tissue-specific resident multipotent stem cells are characterized by profound self-renewal capacity, which allows them to maintain lifelong homeostasis of mature tissues in the absence of disease or injury.
Although a thorough discussion of each tissue-specific stem cell type is beyond the scope of this chapter, a limited description of a few cell types that are most relevant to surgeons is warranted. In the skin, stem cells reside in two general niches, along the hair follicles in the bulge region deep to the sebaceous glands and in the deep interfollicular epidermis.32 The follicular bulge cells proliferate and form the hair shaft as it grows and may contribute to epidermal regeneration after trauma or injury. The deep interfollicular epidermal cells migrate upward to replenish the layers of the epidermis during normal homeostasis of the epidermis, a process that replaces all skin cells every 3 to 4 weeks. In the small intestine, a group of proliferative cells resides at the base of the crypts and send differentiating cells upward to repopulate the mature gut epithelium, with rapid turnover every 4 to 5 days. It is clear that intestine-specific stem cells exist, but the lack of specific antigens for cell isolation has made precise identification of the putative intestinal stem cell elusive, and the structure of the intestinal stem cell compartment remains controversial.33 Interestingly, not all adult organs with regenerative potential depend on stem cell proliferation. The liver and pancreas appear to regenerate through proliferation of adult cells.34,35 In the heart, resident stem cells have limited regenerative potential and have not been shown to engraft when administered exogenously after myocardial injury.36 More experimental work is needed before these populations of tissue-specific resident stem cells can be effectively exploited for regenerative medicine applications.
Adult Multipotent Stem Cells
Multipotent cells exist in several reservoirs in the adult and retain the ability to form many different cellular lineages (Fig. 8-2). Although the differentiation potential of these cells is not as complete as ESCs or induced pluripotent stem cells, their relative abundance and ease of isolation from adult patients establishes adult stem cells as a highly relevant cell type for regenerative medicine applications. Accordingly, adult multipotent stem cells have been a focus of intensive research efforts over the past several decades.
< div class='tao-gold-member'>
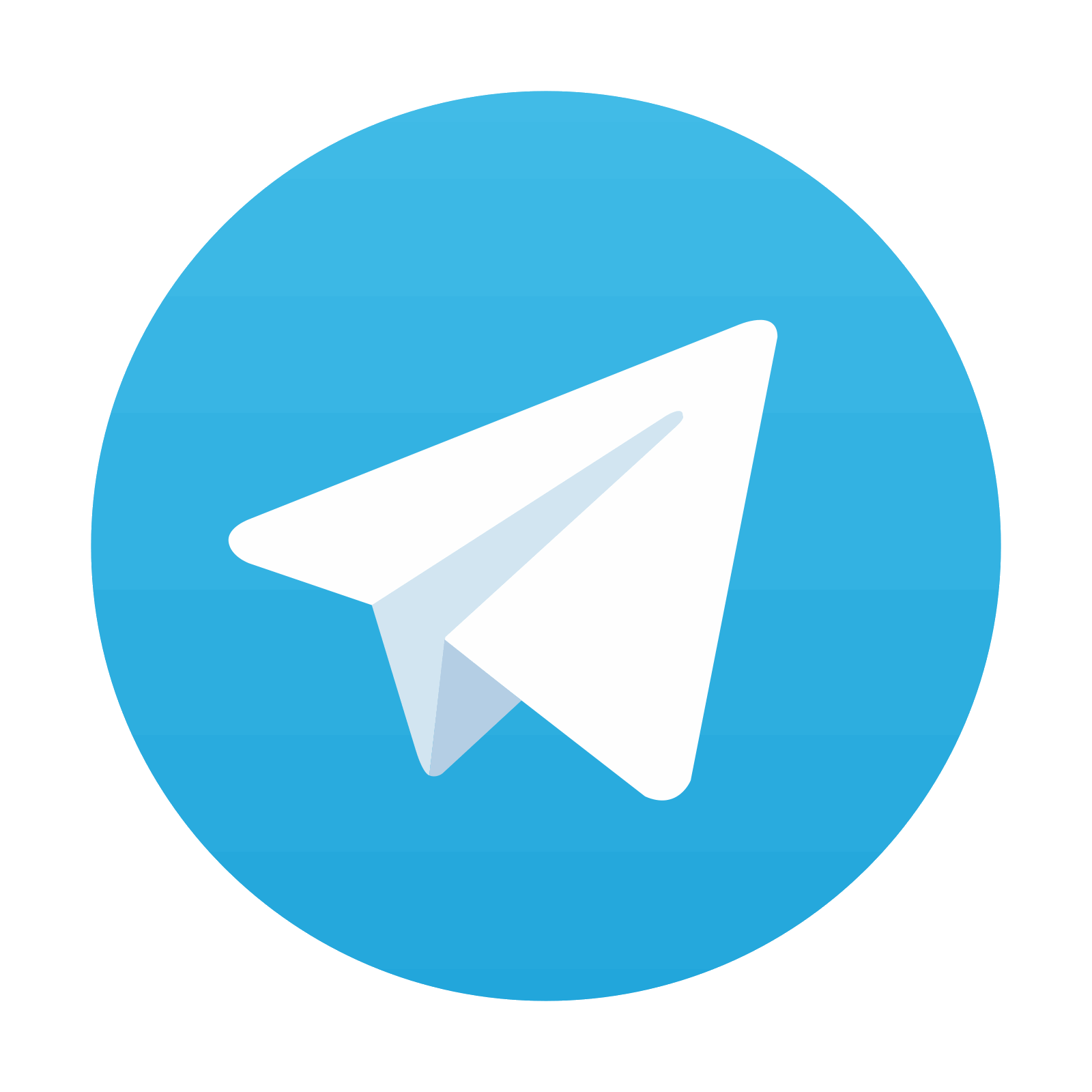
Stay updated, free articles. Join our Telegram channel
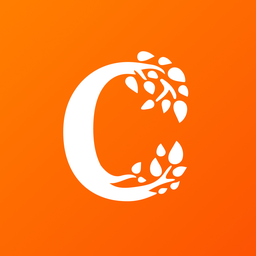
Full access? Get Clinical Tree
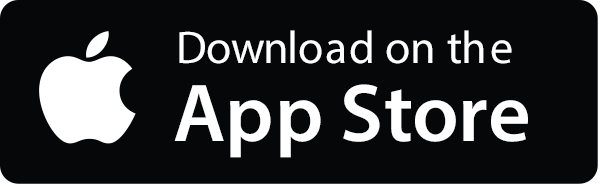
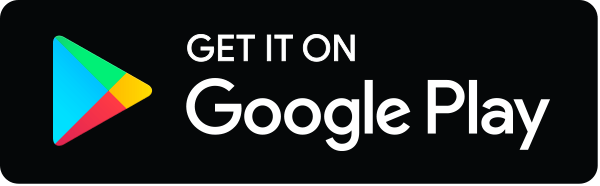