Objective
The study objective was to evaluate whether a novel global position system (GPS)-like position-sensing technology will enable accurate co-registration of images between imaging modalities. Co-registration of images obtained by different imaging modalities will allow for comparison and fusion between imaging modalities, and therefore has significant clinical and research implications. We compared ultrasound (US) and magnetic resonance imaging (MRI) scans of carotid endarterectomy (CEA) specimens using a novel position-sensing technology that uses an electromagnetic (EM) transmitter and sensors mounted on a US transducer. We then evaluated in vivo US-US and US-MRI co-registration.
Methods
Thirteen CEA specimens underwent 3.0 Tesla MRI, after which images were uploaded to a LOGIQ E9 3D (GE Healthcare, Wauwatosa, WI) US system and registered by identifying two to three common points. A similar method was used to evaluate US-MRI co-registration in patients with carotid atherosclerosis. For carotid intima-media thickness (C-IMT) measurements, 10 volunteers underwent bilateral carotid US scans co-registered to three-dimensional US maps created on the initial visit, with a repeat scan 2 days later.
Results
For the CEA specimens, there was a mean of 20 (standard error [SE] 2.0) frames per MRI slice. The mean frame difference, over 33 registration markers, between MRI and US scans for readers 1 and 2 was −2.82 ± 19.32 and 2.09 ± 14.68 (mean ± 95% CI) frames, respectively. The US-MRI intraclass correlation coefficients (ICCs) for the first and second readers were 0.995 and 0.997, respectively. For patients with carotid atherosclerosis, the mean US frames per MRI slice (9 [SE 2.3]) was within range of that observed with CEA specimens. Inter-visit, intra-reader, and inter-reader reproducibility of C-IMT measurements were consistently high (side-averaged ICC >0.9).
Conclusion
Accurate co-registration between US and other modalities is feasible with a GPS-like technology, which has significant clinical and research applicability.
All modalities used for imaging atherosclerosis have inherent advantages and disadvantages. For example, magnetic resonance imaging (MRI) provides highly detailed, structural, and compositional information but suffers from relatively long study times and poor temporal resolution. In contrast, ultrasonography provides real-time imaging with excellent temporal resolution, but image quality can be variable. Combining imaging modalities could theoretically allow their strengths to complement each other and overcome their individual limitations. For example, the portability and immediate availability of real-time data from ultrasonography can be combined with highly detailed, anatomic information from MRI or computed tomography (CT) scans.
A key step for accurate comparisons between modalities involves co-registration, or matching anatomic features between imaging modalities. A novel advance in ultrasound (US) technology, namely, real-time electromagnetic (EM) tracking of the US probe location and orientation, may now allow semiautomated registration between US and other imaging modalities. However, this technology has limited validation.
Therefore, we pursued the validation of this novel real-time EM tracking feature now available in a vascular US system and compared the reliability of its co-registration system with manual registration. We first evaluated ex vivo co-registration between US and MRI using a set of carotid endarterectomy (CEA) surgical specimens and then, in pilot analyses, evaluated in vivo co-registration of US-US and US-MRI.
Materials and Methods
All experiments were approved by the institutional review board.
Ex Vivo Co-Registration
Sample
Atherosclerotic plaques ( n = 13) were obtained after CEA. Specimens were acquired 1 to 3 hours after surgical resection and preserved in phosphate-buffered saline/50% glycerol and stored at −20°C. This preservation method maintains the ultrastructural properties of the carotid plaque.
Sample Preparation
Immediately before use, specimens were dialyzed for 24 hours against phosphate-buffered saline to remove the glycerol. Samples were then embedded in 3% [weight (grams)/volume (milliliters)] low-melting agarose gel (FMC Bioproducts, Philadelphia, PA). The agarose gel solution was created by adding distilled water to agarose gel powder and heating the mixture to 60°C. Before pouring the solution over tissue samples, vacuum suction was applied to the solution. We have found that application of vacuum suction to the solution after mixing minimizes the presence of air bubbles, which are hyperechogenic on US and interfere with US imaging. Once tissues were embedded, the solution was allowed to cool to room temperature before imaging.
Agarose gel was chosen as a support matrix for several reasons: (1) to minimize damage from tissue manipulation; (2) to preserve spatial arrangement of the morphologic features for comparisons between imaging modalities; (3) to minimize chemical interactions with biological tissue, which may interfere with MRI and US imaging; and (4) to provide a support medium necessary for US imaging. Plastic pipette tips were embedded near one end of the CEA sample to provide a non-anatomic reference point.
Magnetic Resonance Imaging
MRI scans were acquired after the CEA samples were embedded in 3% agarose gel in 15-mL culture tubes. These tubes were placed in a custom-built holder designed specifically for acquiring tissue MRI scans. Specimens were imaged using a 6-cm phased array 4-channel carotid coil (Pathway Med Tech, Redmond, WA) on an Excite 3.0 Tesla MRI scanner (GE Healthcare, Wauwatosa, WI). Serial axial proton-density weighted (PDW), T1-weighted, and T2-weighted images were acquired (2-mm slice thickness, matrix 512 × 512, field of view 100 × 100 mm) using a fast-spin echo sequence providing 8–31 slices depending on tissue size with an in-plane resolution of ∼0.195 mm. Correction algorithms adjusted for magnetic field strength gradients across the sample image.
Real-time Three-Dimensional US
After MRI, each of the 13 CEA tissue samples embedded in agarose was extruded intact with the agarose column from its culture tube. The column was then transferred to a plastic box (10.5 × 12.5 × 4.0 cm), and additional 3% molten agarose was added to form an agarose bed prepared as described under the sample preparation section. This procedure was performed to provide adequate surface contact for the US probe. Samples were imaged with a LOGIQ E9 US system (GE Healthcare) using a “free-hand 3-dimensional scanning with EM field sensors” approach. This approach used a mid-range DC magnetic transmitter to generate a weak magnetic field. Position sensors attached to a two-dimensional vascular US probe sensed this field and recorded the probe position through an in-built circuit system, which functioned similar to a global position system (GPS), thereby allowing the computer to know the position and orientation of the transducer and allow it to reconstruct three-dimensional volumes.
Automated Co-Registration
The PDW sequences of the MRI of a given CEA sample were uploaded from a CD-ROM to the US system, and manual marking of two to three features was performed between the MRI and a real-time B-mode US scan. The PDW sequence was selected over T1- and T2-weighted sequences because the PDW sequences were found to have the best image quality (reduced noise, reduced motion artifacts, reduced blurring, and sharp edges). Common features used for initial marking included the plastic marker tip, calcifications, and, if present, the “bifurcation.” After two to three common points between the MRI and the real-time US scans were marked, the US instrument ran an algorithm to create a transformation matrix, which mapped the two-dimensional probe position to a multiplanar reconstruction of MRI scans. The real-time US scan was now registered to the MRI scans, such that movement of the US probe resulted in movement through reconstructed MRI scan planes, displayed next to the US scan.
Validation of US Co-Registration by Manual Methods
All image data were exported in Digital Imaging and Communications in Medicine (DICOM) format to CD-ROMs for offline analysis. Image sets were loaded onto a free DICOM viewer. Each image in a set had three components: (1) a US image; (2) a US frame number that identified the order of the image in the series; and (3) an MRI scan co-registered such that several US frames in the sweep would have the same MRI scan (ie, more US frames than MRI slices) ( Figure 1 ).

Initially, only the US image was blinded out of the three components, and two readers jointly identified two to three features on the MRI scan per CEA tissue sample. The US frame number for a feature and a description of the feature were noted. The total number of MRI scans per tissue sample was also noted.
After all features were identified, the readers independently loaded the image sets in a pre-randomized order unique to each reader, and now they were blinded to the US frame number and MRI scan and unblinded to the US image. Each reader identified the previously described MRI features on the US images. After a feature was satisfactorily identified on a US image, the US frame number was unblinded and recorded as the manually registered frame for that feature.
In Vivo Co-Registration
Reproducibility of C-IMT: US-US Co-Registration
We then examined application of the co-registration technology for improving the reproducibility of carotid intima-media thickness (C-IMT) measurements. Healthy individuals ( n = 10) without coronary heart disease, stroke, peripheral vascular disease, hypertension, or current smoking were invited to participate. All volunteers underwent bilateral US scans of the common carotid artery (CCA) for C-IMT measurements on a LOGIQ E9 US machine. Scans were acquired using traditional methodology and the GPS-like technology. Repeat scans using both methods were performed 2 days later. Neither the traditional method nor the GPS method was electrocardiography (ECG) gated.
The traditional method involved the use of Meijer’s arc for standardizing the probe angle. Volunteers were positioned supine with the neck slightly hyperextended and rotated ∼30 degrees from midline contralateral to the side imaged. The angle on the Meijer’s arc was recorded on the initial visit, and the same angle was used for imaging during the return visit.
For the GPS method, a three-dimensional US sweep of the CCA was performed on the initial visit to create a three-dimensional US map, which was stored. For measurement of C-IMT, both during the initial and return visits, real-time US scans were obtained and co-registered to the three-dimensional map with the GPS-like technology ( Figure 2 ). Semiautomated feature registration using co-registration of corresponding planes followed by point-to-point co-registration (ie, plane-point method) was performed between the real-time US and the three-dimensional map. The plane containing the carotid bifurcation became the internal registration plane for each subject. The carotid bifurcation served as the registration region.

Once the plane-point co-registration was completed during the initial visit, point markers (ie, GPS markers) were placed on the co-registered real-time US scan and saved along with the three-dimensional US map. These markers, which appeared as green crosses when the scan plane was aligned with the markers and as red or blue boxes when the scan plane was directed away from the markers, were used to identify the scan plane/image sequence where the C-IMT measurements will be made. After the plane-point co-registration was completed on the return visit, the GPS markers were loaded from memory, enabling the operator to return to the location of the scan plane where the C-IMT measurements will be made.
All scans were de-identified and exported in DICOM format for offline processing on a workstation equipped to measure C-IMT (Carotid Analyzer, Medical Imaging Applications LLC, Coralville, IA). The C-IMT measurements took place approximately 2 months after image acquisition. For each side, the mean C-IMT value of the far wall of the distal CCA over a 1-cm length was measured, and the combined average of the right and left sides was used for our analysis. Two trained readers blinded to method and to visit order performed the measurements. During the analysis, the readers noted that the quality of the image sequences varied, and they performed a post hoc, blinded, subjective image quality assessment.
US-MRI Co-Registration in Patients with Known Carotid Atherosclerosis
To test US-MRI co-registration in a clinical setting, we invited a subset of individuals ( n = 3, 6 carotid arteries) participating in an ongoing trial ( ClinicalTrials.gov NCT00860184 ) for which Baylor College of Medicine is one center to participate in an ancillary study. The trial enrolls and follows asymptomatic subjects with 50% to 79% carotid artery stenosis in whom a carotid MRI is performed at baseline. The ancillary study that added three-dimensional US imaging was approved by the trial principal investigator.
MRI was first obtained using sequences specified by the trial protocol. The PDW MRI series obtained over an 8-cm length of carotid artery centered on the bifurcation of the carotid artery (2-mm slice thickness, 40 slices total) was then exported in DICOM format and uploaded to a LOGIQ E9 US system. For the US scan, we positioned the subjects similarly to that used for MRI to achieve in-plane registration.
The co-registration method between real-time scans and uploaded MRI scans was similar to that used for ex vivo co-registration between US and MRI. Briefly, real-time US scans were obtained and co-registered to the uploaded MRI scan using semiautomated feature registration of corresponding planes followed by point-to-point registration ( Figures 3 and 4 ). Validation by manual methods was conducted as described for CEA specimens; we used the disappearance of the flow divider as a common feature to evaluate registration between the imaging modalities.


Statistical Methods
The MRI slices per sample, US frames per sample, and US frames per co-registered MRI slice were described using Stata 11 (Stata Corp, College Station, TX). Absolute agreement between US co-registered frames and manual co-registration were determined by calculating intraclass correlation coefficients (ICCs) using a two-way random effect analysis of variance (ICC [2, 1] method) model to test strength of absolute agreement. ICCs were also calculated using a multilevel hierarchic model with mixed effects, which adjusted for variations within each CEA specimen. Bland–Altman plots were generated to examine for biases inherent to registration methods used and to evaluate 95% limits of agreement between mutual MRI-registered and independent US-registered frames.
For the C-IMT measurements performed for US-US co-registration, the ICC [2, 1] method was used to determine inter-visit repeatability and inter- and intra-reader reproducibilities. Bland–Altman plots were also generated to examine for biases inherent between methods. For the US-MRI co-registration conducted in patients with known carotid atherosclerosis, the difference in frames for common features observed on US and MRI was noted and described.
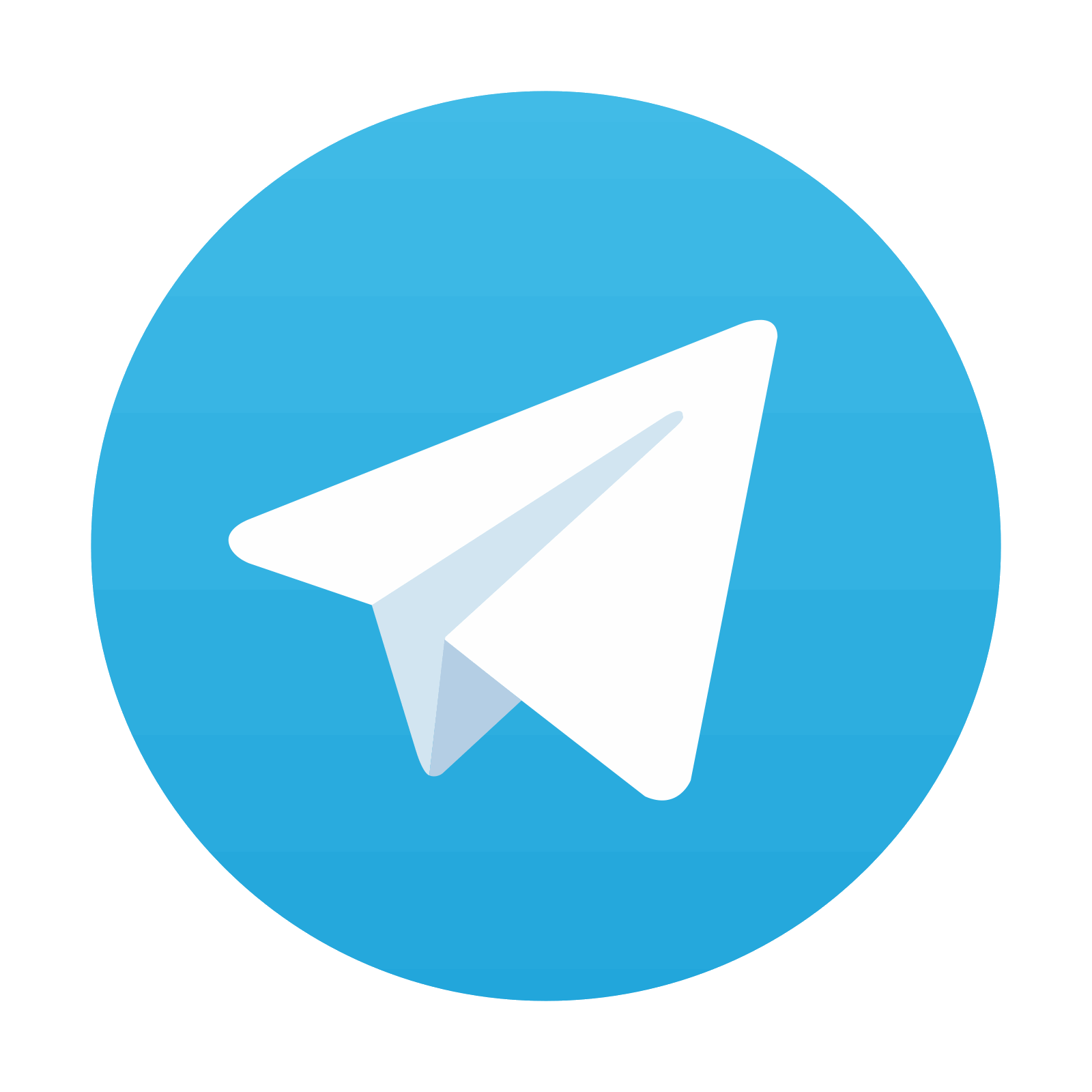
Stay updated, free articles. Join our Telegram channel
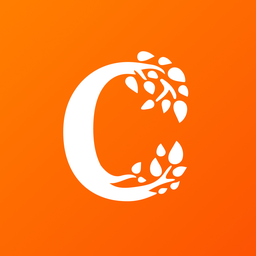
Full access? Get Clinical Tree
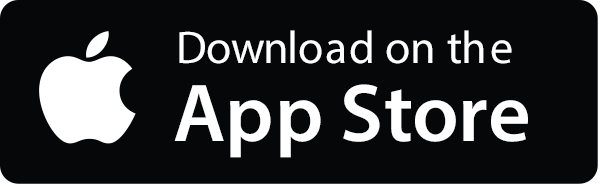
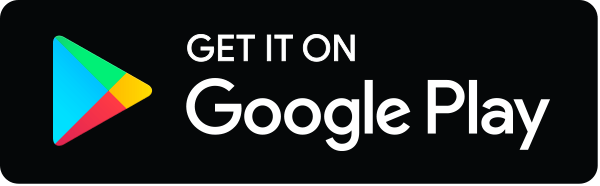