Background
Cardiosphere-derived stem cell (CDC) transplantation can improve global left ventricular ejection fraction (LVEF) after myocardial infarction (MI). The aim of this study was to examine the effects of CDC transplantation on regional function and dyssynchrony after MI.
Methods
Two million rat CDCs ( n = 7) or phosphate-buffered saline ( n = 7) was injected into the infarct regions of Wistar Kyoto rats. Infarct size and CDC localization were evaluated by positron emission tomography ( n = 7). Two-dimensional and strain echocardiography were performed at 1 and 4 weeks after MI. LVEF, circumferential strain, and time to peak circumferential strain were measured in the basal and apical short-axis views. Dyssynchrony was defined as the maximal difference of time to peak circumferential strain of opposing segments in each short-axis view. Engraftment was measured by quantitative polymerase chain reaction.
Results
Positron emission tomography revealed that infarct size was 15.4 ± 3.6% of the left ventricle and that CDCs were localized to the infarct and border zone. CDC transplantation improved mean LVEF (45 ± 8% to 52 ± 7%, P = .02), mean circumferential strain (−7 ± 2% to −10 ± 1%, P = .02), and mean dyssynchrony (45 ± 10 to 28 ± 11 m sec, P = .04) of the infarct/peri-infarct zone from 1 to 4 weeks after MI, despite CDC engraftment of only 2.4 ± 3%. In contrast, mean LVEF (48 ± 5% to 40 ± 4%, P = .03) and mean circumferential strain (−8 ± 2% to −7 ± 1%, P = .02) of the infarcted region deteriorated, with no significant change in dyssynchrony (42 ± 12 vs 46 ± 13 m sec, P = .60) in the saline group during the same time period. Change in LVEF was correlated with change in circumferential strain ( r = −0.8, P = .002) and dyssynchrony ( r = 0.6, P = .02) of the infarct/peri-infarct region at 4 weeks after MI.
Conclusions
CDC therapy enhanced LVEF by improving circumferential strain and decreasing dyssynchrony of the infarct/peri-infarct region at 4 weeks, but not 1 week, after MI. Cellular resynchronization therapy using CDCs may be an alternative to traditional electrical cellular resynchronization therapy in post-MI dyssynchrony.
Myocardial infarction (MI) is an important cause of heart failure. Left ventricular dyssynchrony, frequently observed in heart failure, is a predictor of poor outcomes. Cardiac resynchronization therapy can reduce symptoms, induce reverse remodeling, and improve survival, but patients with ischemic cardiomyopathy often do not respond to this therapy. Stem cell transplantation is a novel therapy that offers the potential of regenerating myocardium and improving mechanical synchrony after MI.
Autologous cardiosphere-derived stem cell (CDC) therapy is currently in phase 1 clinical trials in the United States (Cardiosphere-Derived Autologous Stem Cells to Reverse Ventricular Dysfunction; http://clinicaltrials.gov/ct2/show/NCT00893360 ). CDCs are progenitor cells derived from monolayer outgrowth of heart tissue and are composed of subpopulations of cells with markers of cardiac progenitor cells (c-kit + /CD90 − ), mesenchymal stem cells (c-kit − /CD105 + , CD90 + ), and endothelial cells (c-kit − /CD34 + ) that together have a synergistic effect on cardiac regeneration. Experimental studies have revealed that on transplantation into infarcted myocardium, CDCs secrete paracrine factors, differentiate into endothelial cells or cardiac myocytes, and improve left ventricular ejection fraction (LVEF). Because paracrine factors can affect global cardiac function, it is essential to determine the contribution of improved regional function of the infarcted region to increase in LVEF after CDC transplantation after MI. In this study, we investigated the contribution of regional cardiac function and mechanical dyssynchrony in the recovery of LVEF after CDC transplantation in a syngeneic rat model, because it permits cell tracking by positron emission tomography (PET), detailed assessment of cardiac function by echocardiography, and quantification of engraftment by quantitative polymerase chain reaction (PCR). We hypothesized that CDC therapy would improve function by inducing reverse remodeling of the infarcted region. Our results indicate that despite low levels of CDC engraftment, circumferential strain and dyssynchrony improved in the infarct/peri-infarct region and were correlated with increase in LVEF 4 weeks after MI.
Methods
Isolation of CDCs and Tissue Culture
CDCs were isolated from the hearts of male, 3-month-old, Wistar Kyoto rats (Harlan Laboratories, Inc., Indianapolis, IN), as described previously. Briefly, small pieces of myocardial tissue (explants) derived from young male rats were placed on fibronectin coated dishes. In the following days, cells exited the explants and formed an adherent monolayer on the dish surface with phase-bright cells on top. These cells were harvested with mild enzymatic digestion and transferred to d -poly-lysine–coated dishes, where they formed three-dimensional structures called cardiospheres, which were enriched in cardiac progenitors. Cardiospheres were subsequently harvested and grown as monolayers in fibronectin-coated flasks; these cells are called CDCs. CDCs were cultured in Iscove’s modified Dulbecco’s medium (containing 4.5 g/L glucose, 20% fetal bovine serum, 10% glutamine, 10% penicillin/streptomycin, and 0.1 mmol/L mercaptoethanol) and expanded to three passages before transplantation.
Animal Surgery: Cell Injection
Rats were chosen for these studies because they permit detailed assessment of cardiac mechanics by echocardiography, cell tracking by in vivo PET, and quantification of engraftment by PCR. We used Wistar Kyoto rats, which are genetically identical, and performed gender mismatched transplantation: CDCs derived from male rats were injected into female rats to permit quantification of engraftment by quantitative PCR, for the male-specific sex-determining region Y ( SRY ) gene.
Female Wistar Kyoto rats weighing 180 to 200 g were used. Animals were intubated; anesthesia was induced with 4% isoflurane inhalation and maintained with 2% inhalation. The heart was exposed through a left lateral thoracotomy, MI was induced by permanent ligation of the middle portion of the left anterior descending coronary artery using a 5.0-mm silk suture (Ethicon Endo-Surgery, Somerville, NJ) and animals were randomly assigned to two groups: the control group ( n = 7) and the cell group ( n = 7). Immediately after left anterior descending coronary artery occlusion, 100 μL of phosphate-buffered saline (PBS) (in the control group) or 2 million rat CDCs (in the cell group), suspended in 100 μL of Ca 2+ -free and Mg 2+ -free PBS, were injected intramyocardially into two sites of the infarct region, using a 28-gauge needle. The chest was then closed, and animals were allowed to recover.
PET
In vivo PET of 18 F-fluorodeoxyglucose–labeled rat CDCs and myocardial perfusion ( 13 NH 3 ) were performed in a separate group of animals ( n = 7) to characterize the infarct model and examine CDC localization after transplantation. See the supplemental data section for details of PET and data analysis.
Echocardiography
We performed echocardiography in 10 noninfarcted rats and in infarcted rats at two time points: 1 and 4 weeks after MI. We chose the 1-week time point because of poor image quality early after MI due to the presence of a left-sided pneumothorax. Rats were anesthetized with 1.5% isoflurane (using a nose cone) for the duration of imaging; heart rate was monitored, and body temperature was maintained at 37°C during image acquisition. We used a 14-MHz transducer coupled to a Vivid 7 machine (GE Medical Systems, Milwaukee, WI). Two-dimensional long-axis images were used for ejection fraction measurements (calculated as the difference between end-diastolic and end-systolic volumes normalized to end-diastolic volume, expressed as a percentage). Subsequently, an apical short-axis image (distal to the papillary muscles, including the infarcted region) and a basal short-axis image (basal to the infarcted region, at the level of the papillary muscles) were acquired. LVEF was calculated using the area-length method. The adjustments of the sector width for this transducer resulted in a maximum frame rate of 90 to 100 frames/sec.
Circumferential Strain
Initially, we analyzed radial and circumferential strain for measurement of dyssynchrony. In the first two animals, we noted significant variability in peak amplitude of strain and time to peak strain in the radial direction. Hence, we restricted our analysis to circumferential strain. Additionally, circumferential strain has been shown to be a more dynamic parameter and indicative of dyssynchrony in an experimental model. Furthermore, the feasibility and reproducibility of circumferential strain in small animals are superior to those of longitudinal and radial strain. Apical long-axis views in rodents are challenging to obtain repeatedly, reducing the feasibility of longitudinal strain. Similarly, because of sampling issues, radial strain correlates poorly with reference standards and appears more variable in validation studies. Circumferential and radial strain contribute to overall changes in chamber volume over the cardiac cycle more so than longitudinal strain and thus would better reflect changes in ejection fraction in our model.
Image processing was performed offline. We used the speckle-tracking algorithm in the EchoPAC SW 7.1.1 version PC workstation (GE Medical Systems) to measure circumferential strain. On the basis of the two-dimensional short-axis images, the end-systolic and end-diastolic time points were defined; subsequently, three different cardiac cycles from each acquisition were selected for further analysis. Briefly, after selecting the cardiac cycle, the software prompts the operator to apply a region of interest in a “click-to-point approach” to delineate the endocardium. Subsequently the software automatically defines an epicardial and midmyocardial line and processes all frames of the selected cardiac cycle. Then, the software automatically outlines six segments per short-axis view. The circumferential strain value was calculated for each segment. Circumferential strain values are negative values; as contraction progresses, the circumferential strain values become more negative. Segments with poor-quality tracking were excluded from the analysis. For the apical short-axis views, we identified the segment with the lowest circumferential strain value at 1 and 4 weeks after MI for each animal, and we refer to it as regional strain of the infarcted region. Mean circumferential strain was defined as the average strain of all segments in a particular short-axis view. Intraobserver and interobserver variability analyses were performed using five randomly selected studies. For intraobserver analysis, measurements of the five selected studies were repeated on two separate occasions by the same observer. Interobserver analysis was performed by two independent observers who were blinded to the results of each other.
Mechanical Dyssynchrony Evaluation
In all animals and for all myocardial segments, the time to peak circumferential strain was calculated using GE analysis software. For each animal, mechanical dyssynchrony was assessed for the apical and basal short-axis acquisitions at 1 and 4 weeks after MI by measuring intersegmental mechanical delay. More specifically, the time delay between opposing myocardial segments of the basal and the apical short-axis views was calculated separately, and the higher measured delay was used as a measure of mechanical dyssynchrony for the base and apex, respectively. Three different cardiac cycles were analyzed, and the values were averaged.
Real-Time Quantitative PCR for Quantification of Engraftment (Quantitative PCR for Rat SRY Gene)
Animals from the cell-injected group were sacrificed 4 weeks after CDC transplantation; hearts were explanted, weighed, and homogenized. Injection of CDCs harvested from male rats into female recipients permitted the use of the male-specific SRY gene, located on the Y chromosome, as a target for quantitative PCR. Because there is only one copy of the SRY gene per cell, the number of copies of the SRY gene (determined by quantitative PCR) in the recipient heart corresponds to the number of transplanted cells (see supplemental methods ).
Statistical Analysis
Values are reported as mean ± SD. Paired t tests were used for within-group comparisons of the echocardiographic measurements at 1 and 4 weeks after MI. Spearman’s correlation coefficient was used to assess the correlations of the echocardiographic parameters. Independent-samples t tests were used to compare the ejection fraction values of the two groups at 1 and 4 weeks after MI. P values < .05 were considered statistically significant. Mean differences, standard errors, 95% confidence intervals, and t tests were performed for calculation of interobserver and intraobserver variability.
Results
Characterization of Infarct Model by PET
The mean infarct size in this model was 15.4 ± 3.6% of the left ventricle. Intramyocardial CDC injection resulted in cell localization in the infarct and border zone ( Figure 1 ).

Noninfarcted Baseline Echocardiographic Parameters
Baseline echocardiographic parameters were measured in noninfarcted rats ( n = 10) and are shown in Table 1 . Mean circumferential strain at the apex ( n = 9) and base ( n = 9) was similar. The mean mechanical delay of the base and apex was 12.1 ± 5.6 m sec and 9.4 ± 2.5 m sec, respectively ( P = NS).
Parameter | Base | Apex | P |
---|---|---|---|
Mean circumferential strain (%) | −22.6 ± 3.1 | −23.0 ± 5.7 | .866 |
Mechanical delay (m sec) | 12.1 ± 5.6 | 9.4 ± 2.5 | .244 |
Heart rate (beats/min) | 283 ± 43 | ||
Ejection fraction (%) | 76.2 ± 6.7 | ||
Left ventricular end-diastolic volume (μL) | 189 ± 65 | ||
Left ventricular end-systolic volume (μL) | 44 ± 19 |
CDC Transplantation Improved LVEF After MI
There was no significant difference in LVEF between the two groups of animals 1 week after MI ( P = .30), indicating that all animals experienced a similar ischemic insult. However, 4 weeks after MI, there was a significant increase in LVEF in the cell group (44.6 ± 7.6% vs 51.5 ± 7.4%, P = .02) and a significant decrease in the control group (47.8 ± 4.8% vs 40.2 ± 4.2%, P = .02) ( Figure 2 A). Left ventricular end-systolic volume and end-diastolic volume did not differ significantly among the control and cell groups at 1 week after MI (end-systolic volume, 128 ± 13 vs 133 ± 28 μL; end-diastolic volume, 248 ± 24 vs 240 ± 38 μL; P = NS). At 1 month after MI, end-systolic volume was 227 ± 64 μL in the control group (a 76.5% increase; P = .011) and 183 ± 80 μL in the cell group (a 37.6% increase; P = .129). Left ventricular end-diastolic volume was 386 ± 111 μL in the control group (a 55.8% increase; P = .018) and 365 ± 117 μL in the cell group (a 52.0% increase; P = .033).

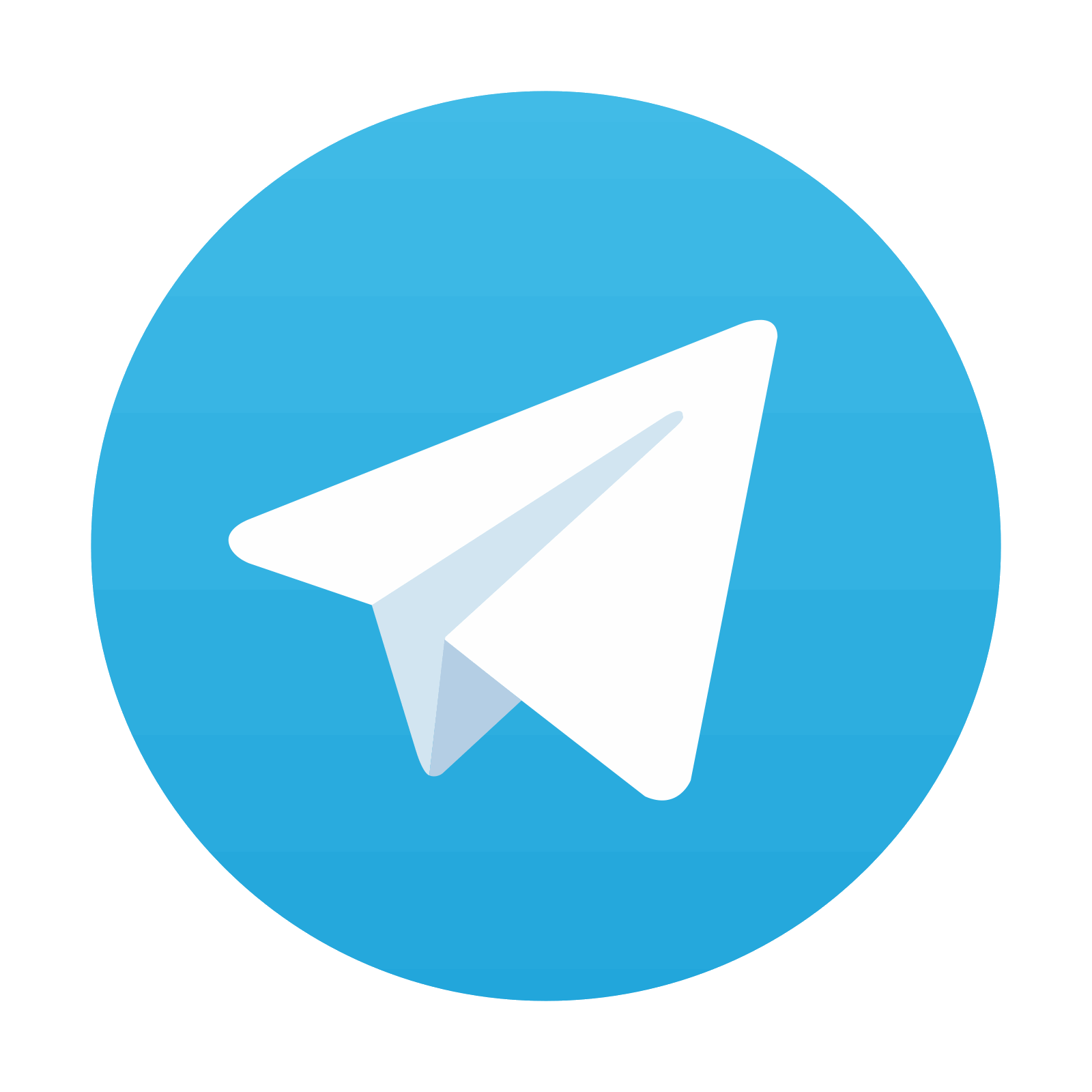
Stay updated, free articles. Join our Telegram channel
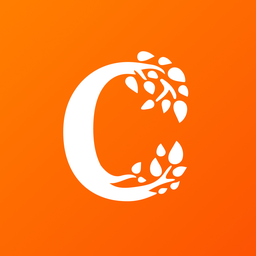
Full access? Get Clinical Tree
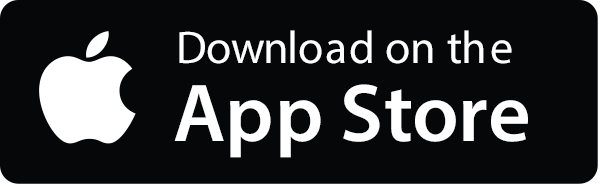
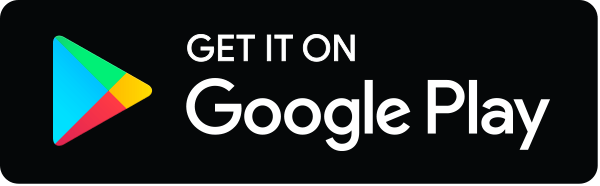
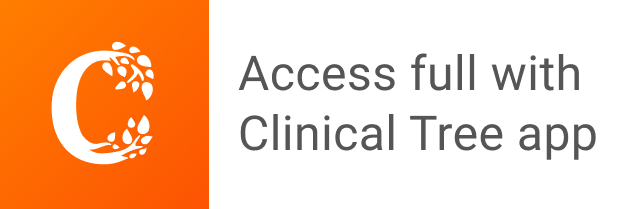