Abstract
Lung function testing in early life can provide information on lung growth and development and can help clinicians in the decision making process. However, the techniques available in infants and preschool-age children are usually not adequately validated for these age groups. Understanding the impact of measurement conditions on the data is essential to achieving valid results. Infant lung function tests are still limited to research settings in highly specialized lung function laboratories and are not suited for the everyday clinical use. In contrast, tests in preschoolers are easy to perform; most of the tests only require minimal subject cooperation and can be undertaken as early as 3 years of age with high feasibility. The main limitations of these techniques are that they are more useful at detecting differences between groups of healthy children and children with lung disease, but they are not specific and sensitive enough to be utilized in individuals in the clinical practice. Interpretation of the results also requires comprehensive understanding of respiratory physiology. There are promising techniques in the focus of pediatric respiratory research in recent years that are likely to improve the clinical utility of lung function measurements during these critical years of lung development.
Keywords
lung volumes, plethysmography, multiple breath washout technique, forced expiratory volume and flow, spirometry, raised volume rapid thoracoabdominal compression, resistance and compliance, single breath occlusion technique, interrupter resistance, specific airway resistance, forced oscillation technique
Introduction
The childhood years represent a very important period of lung growth and development, during which the respiratory system is exceptionally vulnerable to any insult. Measuring lung function in infants and young children presents significant and particular challenges. An ideal lung function test for clinical use should: (1) be easy and quick to perform for both the children and testers even in a busy outpatient setting; (2) have standard operating procedures, guidelines and robust healthy reference data available; (3) discriminate between healthy children and those with respiratory disease with high specificity and sensitivity; (4) be feasible at any age for longitudinal follow-up of individuals with lung disease; and (5) be cheap and widely available to fulfill health care equality.
While the indications, methodology, and interpretation of lung function testing in children above 6 years of age is similar to those in adults, infants and preschool-age children require special technical and safety considerations (these will be discussed below). However, staff with a special attitude, understanding, patience, and love for the children are essential: child-friendly personnel is one of the main determinants of the success of any lung function test in young children.
Indications and Special Considerations for Pulmonary Function Tests in Infants
Lung function can be measured as early as in the first days of life in infants for both clinical and research purposes. Correctly performed lung function tests during infancy can provide information on lung growth and development, disease status, and progression, and can aid the clinicians in decision making. Most of the lung function tests were originally developed for adults and later modified for the special requirements of infants and young children (e.g., plethysmography and multiple breath washout [MBW]), while others have been designed specifically for infant testing (e.g., single occlusion technique [SOT]). Although commercial devices and validated guidelines are often available, infant lung function testing is not always part of the routine practice of pediatric respiratory laboratories. This is most probably due to the special technical and personal requirements that are essential for successful lung function testing during infancy.
Technical and Staff Requirements
Since all of the lung function tests are performed during quiet sleep or sedation, the appropriate location of the infant testing room is very important for the success of the test. In laboratories where lung function testing is performed routinely as part of the clinical care, the infant study room should be isolated from those for preschool- and school-age children. The room containing the lung function devices and consumables has to be comfortable and large enough to accommodate two examiners, one or two parents (and sometimes siblings), and equipped with everything that is necessary for changing and settling the infant.
Since the visit can take as long as 3 or 4 hours, the procedures and their duration (either clinical or research study visit) should be discussed in detail with the parents prior to the appointment. It is important to consider that many of the mothers may be still breastfeeding at the time of the measurement; therefore privacy has to be provided to the parents, if necessary.
Resuscitation equipment has to be available in the room and, for safety and technical reasons, two skilled examiners have to be present at the time of the testing. If the test is performed during sedation (see later) heart rate and oxygen saturation should be monitored continuously.
Infant lung function testing is technically challenging; therefore the examiners have to be properly trained. They should be able to recognize any technical problems (such as software problems, leak in the system during the test), and it is desirable that they have a basic understanding of respiratory physiology. Since the test is time consuming, there is usually no opportunity to repeat it (especially when performed for research); therefore the real-time quality control (i.e., whether the test fulfills all quality criteria) and interpretation (i.e., whether the results are physiologically meaningful) of the test are crucial.
The infant lung function devices vary in their size and the consumables required. Since the tidal flow and volume during infancy are relatively small, maximum reduction of the dead space is a very important requirement in all lung function equipment. To avoid rebreathing of CO 2 , most of the devices employ a bias flow (usually with medical air, but sometimes with oxygen or special gas mixtures).
In spontaneously breathing infants, all of the lung function tests are done through a facemask in the supine position ( Fig. 11.1 ), which introduces the most common source of error in infant testing: the leak around the mask. Any leak invalidates all infant lung function tests; therefore the size of the facemask always has to be selected carefully and its position checked before and throughout the test. Furthermore, the facemask increases the equipment dead space, and its volume should always be taken into account for corrections. However, mask volume correction is not straightforward as one cannot always predict how much of the mask is occupied by the infant’s face, raising the possibility of overcorrection for mask volume. Unsedated babies sometimes do not tolerate the facemask and wake up every time when the mask is positioned on their face; this is less frequent under sedation.

In unsedated infants, the natural variability of the breathing pattern is significant, especially during rapid eye movement (REM) sleep. Previous studies have shown that during REM sleep breathing frequency is increased and functional residual capacity (FRC) is unstable; therefore if the lung function test is undertaken without sedation, lung function should be measured during deep, non-REM sleep (whenever possible). Non-REM sleep can be recognized by observation of the baby, and a pulse oximeter can sometimes help to detect stable, regular heart rate (as heart rate is usually irregular and fast during REM sleep). Note: monitoring of oxygen saturation is mandatory when infants are sedated for lung function testing and optional for studies conducted during natural sleep. The sleep of preterm babies and neonates is usually superficial, and it is not always possible to differentiate between REM and non-REM sleep phases. Therefore a lung function test in preterm infants and newborns cannot always be undertaken in one sitting and sometimes requires two or three sessions. If more than one lung function test is performed in an infant, the tests that require tidal breathing should be undertaken first while the tests that require a controlled maneuver and may affect the respiratory status (e.g., rapid thoracoabdominal compression [RTC]) should be performed at the end of the visit.
Notes on the time and duration of the tests, and replacement of the facemask between measurements should always be recorded as well as the behavior of the infant (i.e., expiratory noise, snoring, sighs). These comments are useful for the correct interpretation of any infant lung function test.
Lung Function Test in Sedated Infants
Although some lung function tests (typically the ones that require quiet tidal breathing) can be performed in unsedated babies sometimes as late as 1 year of age, there are tests that are difficult or impossible to perform without sedation. Sedation for lung function testing has become less common and has to be considered carefully for either clinical or research purposes. If lung function is measured as part of the clinical care in an infant with ongoing respiratory problems, sedation is more accepted and tolerated by the parents. Collection of reference data in healthy infants can therefore be challenging, although it is required for the meaningful interpretation of any lung function measure in children with respiratory disease. Nowadays, many human research ethics committees hesitate to approve the sedation of healthy children or even children with respiratory disease for research purposes. Sedation is also contraindicated in neonates and preterm babies with immature respiratory control or any disorders that affect the neural system of the infant. Sedation affects the breathing pattern and the FRC of the infants; therefore data collected in unsedated and sedated babies with the same technique cannot be compared directly. However, it has been shown that the sedation has no effect on the activation of the Hering-Breuer reflex ; therefore it can be used safely in techniques such as the SOT or the low-frequency forced oscillation technique (LFOT).
Independently of the purpose of the sedation (i.e., clinical or research test), the exact process and the possible side effects always have to be discussed in details with the parents. It is important to note that even in sedated babies it can take from 15 to 90 minutes to reach quiet sleep that is necessary for the test. Chloral hydrate in an oral dose of 50–100 mg/kg is most frequently used for sedation and usually allows 30–45 minutes for the examiner to perform the lung function test(s). Side effects are extremely rare; however, parents always have to be provided with a phone number to call if they have any concerns after the visit, and they should also be advised not to leave the child unattended during the first few hours.
Indications and Special Considerations for Pulmonary Function Tests in Children
Children older than 2 years of age cannot be sedated for a lung function test; however, their cooperation during the preschool years is limited: they are rarely able to perform complex respiratory maneuvers that are required for lung function tests routinely used in older children and adults. Furthermore, the anatomical and physiological differences in the respiratory system between young children and adults make the interpretation of lung function measures originally developed in adults less straightforward and sometimes meaningless. Common interests of clinicians and researchers have resulted in the development of novel lung function methods and modifications of previously available techniques; therefore nowadays more than half of the children at 3 years of age and 80% of the children between 4 and 6 years of age are able to perform acceptable lung function tests. Similar to adults, spirometry is the most frequently used lung function test in children above 6 years of age, while tests that only require tidal breathing are more favorable in preschool-age children.
Technical and Staff Requirements
A child-friendly environment ( Fig. 11.2 ) with engaged and friendly respiratory technicians or researchers is one of the most important key points for successful lung function testing in preschool-age children. Since children have a short attention span, their engagement throughout the test is crucial and sometimes challenging. The examiner should understand and respect the personality (and the current mood) of the child and should recognize which children will be able to (or will not be able to) perform the lung function test after some encouragement (if the child does not even want to stand on the scale, it is usually a bad sign). In a clinical setting, especially in children with chronic respiratory disease, attempts should always be made to perform the appropriate lung function test(s) even if the failure is predictable. This will make the child comfortable and familiar with the test and can be considered as training for the next occasion. Giving the mouthpiece and nose clip to the child to play with at home can be a useful introduction and may increase testing success on the next visit.

It is always important to take the time and explain the test to the child in understandable language and even involve them in the preparation of the test (e.g., to connect the mouthpiece to the device). Young children can cope with the test better if it is separated into small steps and they have to complete one step at a time. Continuous positive feedback is very important and all the attention of the examiner should be focused on the child throughout the visit. For safety reasons, children should never be left unattended in the respiratory laboratory. Preschool-aged children are very open-minded and creative, they are always ready for some play and magic; therefore (if the examiner is prepared) the visit can be great fun.
Since clinicians are rarely present during the lung function test, technicians should provide them with written or verbal comments on the test when the results are presented. Comments on effort (in spirometry), posture, leak, etc., should always be recorded and taken into account for the interpretation. Bronchoprovocation tests are hardly ever done in children younger than 5 years of age; however, the response to bronchodilator is frequently assessed in both clinical and research settings. The bronchodilator test, its interpretation, and clinical utility will be discussed as a part of the detailed description of the lung function methods later in this chapter (see Measurements of Forced Expiration and Measurements of Respiratory Resistance and Compliance ). Bronchoprovocation tests in children above 5 years of age are discussed in detail in Chapter 12 and therefore will not be included in this chapter.
As a part of the lung function test, information that is expected to influence the interpretation of the lung function results should always be collected, including date of birth, height, weight, sex, environmental data, and relevant medical and family history. The interpretation of the lung function data in adolescents is further complicated by the hormonal changes and their effects on lung growth. Healthy reference values, appropriate for both the measured population and the lung function device, therefore need to be selected carefully. Ideally, the results are presented in terms of z-scores, which take into account both the measured and predicted values as well as the test variability within the population. Reference values collected in multicenter studies are especially useful as they can prove the robustness of the technique in different research and clinical environments. The examiner always needs to investigate the relevance of the reference data and the results should be reported accordingly.
Manufacturers and local distributors should be aware of any specific requirements that are necessary for the clinical testing of infants and young children (i.e., dead space reduction, appropriate calibration device, facemask and filter, etc.) and always provide the users with in-depth training and relevant guidelines. This is especially important when a novel lung function method is introduced for clinical use to respiratory technicians and physicians who are not familiar with the requirements of the test and interpretation of data. The proper introduction of a novel method requires a high level of flexibility from the manufacturer and strong collaboration between researchers and clinicians to ensure the collection of reliable data and meaningful interpretation of the results in a clinical situation.
Measurement of Lung Volumes
Summary
- ▪
The measurement of lung volumes is crucial for the interpretation of lung function
- ▪
Functional residual capacity is the only lung volume that can be measured at any age
- ▪
Vital capacity and its subdivisions can only be measured in cooperative children with spirometry
- ▪
Total lung capacity and residual volume can be determined from the spirometric measures if FRC is known
The measurement of lung volumes in infants and children is crucial for the interpretation of the measures of lung mechanics (resistance and compliance), respiratory flows, and ventilation inhomogeneity, and can provide information on lung growth and development. There are two techniques that were originally developed for adults and later modified for infants and young children: body plethysmography and the gas dilution technique. While in cooperative, older children, a wide range of lung volumes can be determined, infants are not able, and preschool-age children have limited ability to perform complex respiratory maneuvers, therefore, only FRC (the volume of the gas present in the lungs at the end of a tidal expiration) can be determined reliably in the early years of life. The underlying assumptions of the measurement of FRC with plethysmography (FRC p ) or gas dilution technique (FRC g ) are different and therefore they require different devices and techniques; both methods have advantages and limitations over the other. Plethysmography estimates all gas compartments in the lungs irrespectively of whether or not they communicate with the airway opening, whereas in the gas dilution measurements only the communicating compartments are measured. In healthy individuals, the difference between FRC p and FRC g is minimal; however, this can be significant in the presence of air trapping when FRC g can be underestimated. In the recent years, measurement of FRC g with the MBW technique has become popular as it does not require sedation during infancy and it is more feasible in young children than plethysmography.
Whole Body Plethysmography
Summary
- ▪
Measurement of thoracic gas volume (including nonventilating areas)
- ▪
Commercial devices are available for both infants and children
- ▪
Mainly used in infants and cooperative older children as preschool-age children rarely tolerate the measurement
- ▪
Results are unreliable in the presence of severe airway obstruction (FRC is overestimated)
- ▪
Has become less common in infants and preschool-age children with the commercialization of MBW
- ▪
Frequently used in children above 6 years of age
The plethysmographic measurement of thoracic gas volume was first described in 1956 by DuBois and his colleagues ; however, the idea of performing respiratory efforts against a closed airway to measure lung volumes has been known since 1882. Unlike the gas dilution technique, plethysmography measures the total thoracic gas volume, including the air that is not communicating directly with the airway opening. Commercial devices with normative values are available; therefore plethysmography has been used in both clinical and research environments during infancy. Since sedation is usually required for the test in younger children, plethysmographic measurement of FRC has become less frequent in pediatric lung function laboratories. Despite the few studies that reported FRC p in preschool-age children, its use is highly limited below 6 years of age and no robust reference data are available for young children. In research studies, reference values calculated for school-age children have been extrapolated to those between 3 and 6 years of age; this method does not meet criteria for predicting lung function in preschool-age children and cannot be used in clinical practice.
Physiological Principles and Assumptions
The measurement of FRC p is based on Boyle’s law, which describes the relationship between volume and pressure of a given amount of gas within a closed system, that is, in the thorax. The assumption is that if the temperature is constant within the system (isothermal conditions) the compression/decompression of the gas (decrease/increase in volume) will change the pressure, so that the product of pressure (P) and volume (V) is constant (k) at any moment:
PV = k
During an (inspiratory) breathing effort against a closed airway, the changes in P from P 1 to P 2 (ΔP) can be measured, and the simultaneous expansion of the intrathoracic gas (ΔV = V 2 − V 1 ) results in an opposite change in the gas volume in the plethysmograph box (V box ), that is,
Δ V box = − Δ V
Thus, knowing ΔV and from the relative changes in alveolar pressure as measured by P 1 at the airway opening, preocclusion lung volume V 1 can be calculated as
V 1 = − P 1 ( Δ V / Δ P )
ΔV can be measured in the body box in which the subject is enclosed, in different ways: (1) as a volume displacement sensed by a closed-circuit spirometer (volume displacement plethysmograph), (2) via the pressure changes in the closed box (pressure plethysmograph), and (3) by integration of the flow across the box wall (flow plethysmograph). This latter type including correction for the residual pressure in the box (pressure corrected flow plethysmograph) is the most commonly used equipment for measurement of intrathoracic gas volume.
In the actual measurement of thoracic gas volume, the patient is seated (or the infant placed, Fig. 11.3 ) in a tightly closed chamber where the changes in the mouth pressure and volume of the chamber are measured over different conditions. At the beginning of the test, tidal breathing is recorded. At this time, the volume of the gas in the chest is unknown while the alveolar pressure is equal to the atmospheric pressure when there is no airflow (i.e., end of expiration or inspiration). Once the end-expiratory level (EEL) is stable, the airways are occluded with a shutter and the patient generates respiratory efforts against a closed airway. In this new condition, mouth pressure is expected to equilibrate quickly with alveolar pressure (this equilibrium can be delayed in lung heterogeneity ; see also below concerning the limitations of the technique). From the pressure changes at the mouth and the changes in the volume of the airtight chamber, intrathoracic gas volume can be calculated, as described above. In infants, it is best to occlude the airways at the end of inspiration as it is less uncomfortable and more tolerable for the infant. If the occlusion appears at end inspiration, tidal volume (V T ) has to be extracted from the total gas volume when FRC p is calculated.

Since the volume of the plethysmograph is relatively large in children and adults, they are asked to pant during the test with a frequency of approximately 60 breaths/min (1 Hz). This controlled breathing frequency helps the equilibrium of the alveolar and mouth pressures, keeps the glottic aperture open, minimizes the thermal drift, and so improves the quality of the test. However, it is important to note that panting does not reflect the normal conditions within the respiratory system during tidal breathing. In infants, the volume of the chamber can be kept relatively small; therefore tidal breathing is adequate to ensure the quality of the test.
The feasibility of the test in infants depends on the sleep state (usually under sedation) and the stability of the EEL. In preschool-aged children, the feasibility is limited as they do not tolerate the closed chamber and panting against a closed airway. In the studies where FRC p was measured in children between 3 and 7 years of age, the measurements were preceded by a long training session both outside and inside of the chamber (i.e., breathing through the mouthpiece and then mouthpiece occluded, supporting their cheeks, etc.). With this method, the test was successful in nearly 70% of the children. In older children, the success rate is similar to that in adults.
Quality Control, Acceptability Criteria, and Limitations
The installation of the equipment has to be planned carefully as both the infant and child plethysmographs are bulky and not portable. For infants, extra room for the adjustment of the facemask and rapid access to the infant has to be allowed for.
Even if the test appears successful, many factors can influence the quality of the measurement and the interpretation of the results. All plethysmographs require precise calibration on the day of the test, which can be time-consuming. Accuracy also has to be checked at least monthly (usually with a lung model or biological control). Prior to the test, the equipment has to be turned on to allow a sufficient warm-up period. The calibration procedure is equipment-dependent; therefore, the instructions of the manufacturer have to be followed step by step. Once the patient is comfortable inside the plethysmograph, the door is closed for a few minutes to stabilize the thermal conditions. Both the calibration and patient preparation (sedation in infants, training in preschool-age children) are time consuming and require patience from both the technicians and parents (and children). If the time allowed for thermal adjustment is not long enough the measurement is unreliable. In modern plethysmographs, the time that the patient has to spend in the chamber before the test is significantly shorter and this may help to make the test more feasible in young children.
Every occlusion should cover at least two complete respiratory efforts (6–10 seconds) and should be repeated three to five times during the test. FRC p is calculated as the mean (SD) of at least three technically acceptable recordings.
The time required for the equilibrium between the pressures at the airway opening and the alveolar system is highly influenced by the resistance and compliance of the upper airways. Usually, a firm support of the cheeks of the patient with the palms is sufficient to decrease the compliance of the cheeks and adequately measure FRC. In the presence of a severe obstruction, transmission of changes in the alveolar pressure may become too slow to reach equilibrium. In this case the change in airway-opening pressure, ΔP will underestimate the change in alveolar pressure and hence FRC will be overestimated. Since infants are preferential nasal breathers, cheek support is not required for the test; however, after the placement of the facemask the system always has to be checked for leaks.
The steps necessary to ensure the quality of the test are equipment dependent and include high-quality pressure transducers and linear pneumotachographs (in the flow plethysmograph), carefully performed calibration, the measurement of biological controls, sufficient warm-up times, and correction of thermal drift. The general recommendations for standardization of the technique have been published, and specific recommendations for infant measurements are also available (see Suggested Reading ).
Spirometric Measurements of Lung Volumes in Cooperative Children
Once FRC is known, other physiologically and clinically important lung volumes can be measured with a simple spirometer and TLC and RV calculated ( Fig. 11.4 ). The complex respiratory maneuver starts with tidal breathing wearing a noseclip. After a few breaths, the child is instructed to take a deep breath to the maximum inspiratory level and perform a maximum expiration thereafter, and finish with tidal breathing. The volume between the maximal inspiratory and expiratory levels is called vital capacity (VC). Following a maximal expiration there is always an air volume in the lungs that cannot be actively exhaled; this is called the residual volume (RV). TLC is therefore the air volume that the lungs contain at maximum inspiration, i.e., VC+RV. TLC and its subdivisions and their definitions are shown in Fig. 11.4 . FRC, TLC, and RV have clinical relevance and therefore they are the most frequently reported lung volumes in older children and adults.

Gas Dilution Technique
Summary
- ▪
Measurement of FRC g with closed-circuit Helium dilution or MBW technique
- ▪
FRC g only represents the ventilated lung parts that are in communication with the airway
- ▪
This test is more popular than plethysmography as it is relatively easy to perform in unsedated infants and preschool-age children
- ▪
It is less commonly used in children above 6 years of age who are able to cooperate with the plethysmographic and spirometric measurements of lung volumes
- ▪
Commercial devices are available for both infants and children
- ▪
Main limitation is the underestimation of FRC in severe airway obstruction
The measurement of FRC with the gas dilution technique has been employed widely in infants and young children as it only requires tidal breathing. Until recently, closed-circuit helium (He) dilution was the most frequently performed test, while nowadays (since commercial devices for MBW test have been introduced), MBW has become more popular in both infants and preschool-aged children.
Physiological Principles and Assumptions
The use of He to determine lung volumes was first introduced in 1941. Since then, the gas analyzer systems have improved significantly; however, the concept of the test and the method of the measurement have remained nearly unchanged. The basic assumption of the method is that the patient with an unknown lung volume breathes from a reservoir of known volume containing He in a known concentration (usually is around 15%). If the circuit is closed, lung volume equilibrates with He (as indicated by less than 0.02% fluctuation in the He concentration for at least 15 seconds) resulting in a decrease in the He concentration ( Fig. 11.5 ). Since He does not transfer through the alveolar membrane to and from the circulation, the final amount of the He is the same as the initial amount (but distributed in a higher volume):
He I × V reservoir = He F × ( V reservoir + FRC g )

With the commercialization of MBW, the parallel measurements of FRC g and ventilation inhomogeneity have become more popular in both preschool-age children and infants. The details of the MBW technique are discussed in the Measurements of Gas Mixing part of this chapter. Briefly, N 2 washout with pure O 2 is the most common MBW test in preschool-age children. N 2 is already present in the lungs in a concentration of about 80%, which is decreasing with every breath that the child takes of pure O 2 . The concentration of N 2 as well as the tidal flow is recorded throughout the test. (In the commercial devices, the concentration of N 2 is measured indirectly: the level of CO 2 and O 2 are detected in the exhaled air and the quantity of N 2 is calculated secondarily.) FRC g is calculated as the product of the net volume of the inert gas exhaled during the test and the difference between the end tidal N 2 concentration at the beginning and at the end of the test, assuming that FRC was stable throughout the test. FRC can also be calculated for each breath separately. SF 6 is frequently used as a tracer gas in infants to avoid the effect of pure oxygen on the breathing pattern and respiratory mechanics. The value of FRC g is usually lower when extrinsic tracer gases are used (such as He, SF 6 ), since N 2 is also excreted from the tissues, which affects the concentration of N 2 within the lungs.
Quality Control, Acceptability Criteria, and Limitations
The quality control, the acceptability, and the limitations of the MBW test are discussed in the Measurement of Gas Mixing part later in this chapter.
Generally, the main acceptability criteria of the different gas dilution tests are independent of the test gas, and it is always desirable to have at least three recordings with FRC g within 25% from each other; however, two tests within 10% may also be acceptable.
Similarly to plethysmography, the test requires a stable breathing pattern, which is difficult to achieve in unsedated infants and young children. Further complications are caused by the use of the facemask because of the difficulty in controlling leaks around the mask. While bigger leaks are usually identifiable by a quick drop in the concentration of the tracer gas during the washout phase of MBW or throughout the He dilution test, small leaks are difficult to recognize, and the risk of reporting false data increases. If the differences in FRC g between successive tests are bigger than 25%, the examiner should always check the system for leak, which is usually resolvable with the replacement of the facemask. Real-time quality control is therefore of utmost importance during the gas dilution test.
Although the gas dilution tests are relatively easy to perform, FRC g only reflects the parts of the lungs that are ventilated and communicating directly with the airway opening. Therefore true FRC can be underestimated with lung disease; this remains one of the main limitations of the technique.
Interpretation of Lung Volumes in Infants and Young Children
Longitudinal measurement of lung volumes in healthy subjects provides information on lung development and growth; however, this is not necessarily true in children with lung disease. In healthy children, FRC correlates with height ( Fig. 11.6 ) and weight, and it is frequently reported as a normalized value for body weight (mL/kg), especially during infancy. Despite the unstable end-expiratory lung volume (EELV) in neonates and infants, the relationship between body weight and FRC is relatively strong in early life. It is a common observation that there is a difference between plethysmographic and gas dilution estimates of FRC, although this difference becomes relatively smaller with growth in healthy children. Since the normative values reported in older infants and young children are highly influenced by the device and the methodology used for the measurement of FRC, it is recommended to measure FRC in healthy subjects in each laboratory to aid the interpretation of the FRC data in children with lung disease. If reference values are available, the relevance of that given dataset (i.e., same device, similar population characteristics, similar data analysis, etc.) has to be investigated carefully.

Both restrictive and obstructive lung diseases affect the value of FRC directly; however, this effect can be distorted because of the different limitations of the measurement technique. FRC can be underestimated with the gas dilution technique in the presence of air trapping —or overestimated if FRC is measured with the plethysmographic method in severe airway obstruction.
One of the most common reasons for low FRC during infancy is surfactant deficiency in preterm babies (respiratory distress syndrome), which results in atelectasis with low lung volumes and low respiratory compliance. In studies on bronchopulmonary dysplasia (BPD) low FRC has been reported during infancy (in most of the studies FRC has normalized later in life). Lung volumes can also be diminished in conditions that affect the chest wall such as muscular disease or chest wall deformations and in congenital diaphragmatic hernia. Some of the autoimmune diseases cause restrictive pattern in the lungs resulting in lower FRC, RV, and TLC with a marked decrease in diffusion capacity of carbon monoxide (DLCO, see also in the DLCO part of this chapter).
Airway obstruction is the most common mechanism underlying the changes in FRC in both infants and young children. Depending on the severity of the obstruction, dynamic hyperinflation may result in increased FRC and can be detected with both the plethysmography and the gas dilution technique. In contrast, closure of the peripheral airways and the consequent air trapping during expiration does not modify the FRC p , whereas trapped air is not included in the value of FRC g (see explanation above). Bronchiectasis-related increase in FRC, RV, and TLC are often present in older children with CF or primary ciliary dyskinesia (PCD), and the increase correlates with the extent of the bronchiectasis and air trapping on the chest CT.
The measurement of FRC is fundamental for the understanding and interpretation of respiratory mechanics; however, it is important to note that FRC is unstable in infants and also highly variable in preschool-age children; therefore the interpretation of a low or high FRC without other measures of lung function has limited clinical value.
The knowledge of FRC is particularly important in the plethysmographic measurement of airway resistance, which is commonly reported as sR aw (R aw normalized for the FRC); this will be discussed in the Measurement of Respiratory Resistance and Compliance part later in this chapter.
Measurement of Diffusing Capacity of Carbon Monoxide
Summary
- ■
It is called transfer factor of carbon monoxide (T LCO ) in Europe and diffusing capacity (DLCO) in the United States
- ■
It provides information on the rate at which the oxygen is transferred via passive diffusion from the lungs to the circulation
- ■
The diffusion across the alveolar-capillary interface can be impaired by both structural and functional changes of the alveoli or the capillaries
- ■
Except for a few research studies in infants, the measurement of diffusion is only performed in cooperative, older children
- ■
New measurement guidelines using rapidly responding gas analyzers (RGA) and robust reference values for the Caucasian population (over 5 years of age) have recently been published. Commercial devices are available
As a very important part of gas exchange, oxygen has to transfer from the alveoli to the capillaries. Oxygen travels through the alveolar-capillary barrier via passive diffusion and the rate is highly determined by structural and functional properties of this system. However, oxygen transfer through the lungs cannot be determined directly.
Physiological Principles and Assumptions
Carbon monoxide (CO) is a gas perfectly designed to measure diffusion from the lungs to the circulation. As there is no CO in the blood (except in heavy smokers), even at low concentrations, CO transfers from the alveoli to the capillaries driven by the pressure difference between the partial pressure of CO in the alveoli (P ACO ) and the capillaries (P aCO ). CO has an enormous ability to bind with hemoglobin (hb) in the vessels, and hb becomes fully saturated even at very low partial pressures. During this transport, the partial pressure of CO changes in the blood to only a minimal degree. Therefore diffusion is the only limiting factor for CO uptake into the blood and CO has been used to assess diffusion for many decades.
The rate of transfer of carbon monoxide from the alveoli to the pulmonary capillaries is a strong indicator of the efficiency of gas exchange in the lung. This recognition resulted in the development of a new lung function method. Although the measurement of diffusion via CO intake was introduced over 100 years ago, it was first standardized for clinical testing in the late 1950s. DLCO is an indicator of all the main determinants of diffusion such as the area and thickness of the alveolar-capillary barrier and the diffusion constant of the measured gas. It can be defined as the conductance of CO from the inspired gas from the alveolar space to the hb-binding sites within the capillaries. Since all the technical details of the measurement method have been published in-depth recently (see Suggested Reading ), only the main principles and interpretation of the test are summarized in this chapter.
During the test, after a few tidal breaths, the child is asked to exhale to RV. According to the recently published recommendations, the expiration time can be as long as 12 s. Because of their smaller lung volumes it is easy for children to empty the lungs over 12 s, even in the presence of severe obstruction. Then, the child is instructed to take a deep, quick breath of a gas mixture containing 0.3% CO, a tracer gas (usually 10% He or 0.3% neon), 21% oxygen and the balance as nitrogen. It is assumed that CO reaches the alveolar space instantly and the alveolar concentration of CO changes rapidly by mixing with the gas volume in the lungs (i.e., RV). The volume of CO in the lungs will be a product of the alveolar volume (V A ) and the fractional concentration of the CO in the alveolar space (F ACO ). The volume of the inspired gas should be close to VC (between 90% and 95%). A suboptimal inspiration (i.e., less than 85% of VC) affects the alveolar volume significantly and hence the DLCO values (see below). Since the assumption of the test is that CO reaches the lungs instantaneously, rapid inspiration is an important acceptability criterion in the measurements of DLCO. Once the child reaches full inspiration, the breath should be held for about 10 s. The proper breath-hold is one of the most difficult parts of the measurement of DLCO. Since both increased and decreased blood flow and alveolar surface area affect diffusion, the breath-hold needs to be voluntary and without effort. Müeller and Valsalva maneuvers should therefore be avoided (see below). Following the breath-hold, the child should exhale immediately with a smooth, relaxed expiration. During the maneuver, modern RGA systems can provide continuous monitoring of the concentrations of CO and the tracer gas as well as the volume over time. However, the analysis of the gas is often performed the same “classical” way as in the older systems. The unit of DLCO is traditionally ml.min −1 .mmHg −1 in the United States, while in Europe the SI unit is preferable (mmol.min −1 .kPa −1 ). Changes in both the structure and function of the alveolar-capillary interface can alter the diffusion of CO, making the interpretation of the results less straightforward.
Quality Control, Acceptability Criteria, and Limitations
The technical details of the measurement setting, gas analyzers and quality control criteria are discussed in detail in the newest guideline and they are outside of the scope of this chapter. Once children can cooperate with the respiratory maneuver necessary for the test, the measurement is quick and easy to perform. In small children, when the VC is less than 1.5–2.l, cooperation is not the only limitation; the measurement can be invalid because of the small volumes and technical modifications should be considered.
Physiological increases or decreases in DLCO and conditions that influence the diffusing capacity that are unrelated to the respiratory system make the interpretation of the results difficult. This remains the main limitation of the test and is discussed in detail below.
Interpretation of DLCO
The largest set of reference values for DLCO has been established for the Caucasian population from 4 to 91 years of age. This dataset includes the recently published children-specific reference values (ref Kopman 2011, Kim 2012). When body weight-adjusted dead space correction was applied to the data, the between site differences in the pediatric values were minimized. The main predictors of DLCO were age, height, and sex and z-scores were created for both males and females. Between 4 and 18 years of age, the absolute value of DLCO increases steeply, while DLCO decreases after 30 years of age. Therefore, DLCO should always be reported as z-scores, using the new Global Lung Function Initiative (GLI) equations in Caucasian children. A physiologically relevant change in DLCO was defined as 0.5 z-score or 10% relative change (around 0.3–0.8 mmol.ml −1 .kPa −1 , which is equal to 0.9–2.4 ml.min −1 .mmHg −1 ). Lower limit of normal (LLN) is frequently used to define abnormal lung function and has been defined as below the 5th percentile of the dataset. Since increased DLCO values usually have limited clinically relevance, and the increase is often physiological, an upper limit of normal (ULN) was not established. Adjusting for hb levels had no significant effect on the z-scores, but hb concentration should be considered, especially in patients with anemia. Recommendations for altitude-correction have also been established.
Physiologically, there are factors that affect the value of DLCO and they can complicate interpretation of the test. One of these factors is increased pulmonary blood flow, which can increase the value of DLCO. This occurs in exercise, via the recruitment of new pulmonary capillaries. In patients with lung fibrosis, the exercise-dependent increase in DLCO is usually missing and this characteristic feature of lung fibrosis can aid the diagnosis. Increased intrathoracic pressure, such as the Müeller maneuver, increases the alveolar surface and hence DLCO; therefore it is important that the child should hold their breath with minimal effort during the test. Hormonal changes in women can also affect the measurement; DLCO is greatest just before menses with high intrasubject variability, while girls in their prepuberty are able to produce a more stable DLCO with minimal variation. The lowest value of DLCO is measured on the third day of menses.
Cigarette smoke can decrease DLCO due to the increase in the COhb in the blood, which increases the back pressure of CO. Therefore a smoking history should always be collected from older children and, in the case of regular smoking, they should be asked to refrain prior to the test. Other nonpulmonary diseases can also influence the diffusing capacity. Increased (e.g., polycythemia) or decreased (e.g., anemia) hb levels can either increase or decrease DLCO, respectively. Left-to right cardiac shunts also increase the blood flow and hence DLCO.
Uneven distribution of ventilation-perfusion in the lungs can reduce DLCO. Since CO only reaches the areas of the lungs where there is ventilation, alveolar volume and hence DLCO can be underestimated in severe airway obstruction. When lung emptying becomes nonhomogeneous, the discrimination between the conductive and alveolar gas can be altered and the sample one takes might not be reflective of the alveolar gas compartment. Since diffusion of CO only happens in areas of the lungs where there are both ventilation and perfusion, if perfusion is diminished in an area it can decrease the global value of DLCO. This can be the case with pulmonary emboli or pulmonary hypertension. However, it is well established that when perfusion is limited in a part of the lungs, perfusion can increase in other parts to maintain normal gas exchange. This is why in pneumonectomy (when the cardiac output flows through a single lung and new capillaries are recruited) DLCO does not decrease to 50% of the original value.
DLCO is most informative in interstitial lung disease (independently of the mechanism of fibrosis) and measurement of CO diffusion is one of the most common tests in this patient group. DLCO has also been shown to be useful in lupus erythematosus, scleroderma, sarcoidosis, and other lung disease that affect the alveoli such as alveolar proteinosis. DLCO can be elevated in obstructive lung disease, keeping in mind the limitations regarding severe obstruction as discussed above.
Measurement of Forced Expiration
Measurement of flow and volume during forced expiration from total lung capacity (TLC) is the most frequently used lung function test in clinical practice in both children and adults. After proper training, 50%–80% of preschool-aged children are able to perform acceptable spirometry, but interpretation of the forced expiratory measures is less straightforward than in adults. To further extend the measurement of forced expiration, two techniques have been developed specifically for the use in infants. Since infants are not able to perform a forced expiratory maneuver, flow-volume curve can only be obtained by rapidly applying pressure around the infant’s chest and abdomen with an inflatable jacket during tidal breathing (rapid thoracoabdominal compression [RTC]) or after inflation of the lungs to TLC (raised volume RTC: RV-RTC). These tests still remain the most commonly performed lung function techniques in infants in a clinical setting.
The underlying physiological principles apply for both the infant techniques and the conventional spirometry; therefore they will be discussed together.
Physiological Principles and Assumptions
The basic finding underlying clinical use of the forced expiratory flow volume curve was established in the 1950s when transpulmonary pressure and flow were measured in parallel at isovolumic conditions in adults. Subjects were sitting in a plethysmograph while an esophageal balloon was employed to detect the transpulmonary pressure continuously, and they were asked to breathe at a given lung volume with increasing effort. While at high lung volume the flow increased easily with the driving pressure, at low lung volumes flow could not increase any further even if the transpulmonary pressure increased ( Fig. 11.7 ). This phenomenon is called flow limitation. Following this observation it did not take long to realize that forced flow-volume curves and hence flow limitation can be achieved during forced expiration across a wide range of lung volume (from TLC to RV) without the need to measure transpulmonary pressure. The maintenance of flow limitation is fundamental to the interpretation of forced expiration and this can be difficult at very high and at low lung volumes. Most adults and older children can achieve and maintain flow limitation in the mid-range of lung volumes and this determines the clinical application of spirometry.

When the expiratory muscles are maximally activated at a high lung volume, the pleural pressure is suddenly raised and this rise is transmitted to the alveoli. However, this pressure rise also changes the transmural pressure of the bronchi and tries to decrease their lumen ( Fig. 11.8 ). Thus, both emptying the lung and obstructing the pathway to the airway opening are driven by the same (maximum) expiratory effort. The two opposing processes determine a dynamic equilibrium resulting in flow limitation. If the airways were rigid, the decrease of expiratory flow would occur gradually because of the loss of force that can be generated by the muscles at decreasing lung volumes. The additional drop in flow is the result of the collapsibility of the airways (or certain segments). Flow limitation occurs when, at a given lung volume, the increase in transpulmonary pressure cannot increase the flow any further. Expiratory flow becomes highly turbulent and passes the narrowed airway segment at a very high velocity. The relationship between the flow and volume during a forced expiratory maneuver shows a characteristic shape in healthy subjects ( Fig. 11.9A ). At the very beginning of the maximum expiration the airflow fast reaches its maximum value (called peak expiratory flow) and then turns into a gradual decrease. The progress of lung emptying (the increase in expired volume) is associated with the fall in expiratory flow until the latter stops on reaching the RV. This association between flow and volume during the forced expiratory maneuver is very strong and can be highly reproducible within a subject, provided the starting lung volume and the maximum expiratory effort are consistently maintained in the successive maneuvers. Intuitively, the key issues are (1) how collapsible are the bronchial segments, (2) where is the most collapsible segment (the “choke point”) in the airway tree, and (3) how different are the mechanical properties of the lung regions in the emptying process. Indeed, the diverse patterns of emptying observed in the different lung pathologies (see Fig. 11.9A ) are associated with these structural-functional aspects. Since the lung emptying happens much faster in young children, the measurement of forced expiration does not always provide meaningful clinical information even if the maneuver was performed correctly. Narrowing of airways due to inflammation, mucus production, bronchoconstriction, and airway wall remodeling potentiate the bronchi for the flow limitation to occur at relatively high lung volumes, and loss of the tethering forces in an emphysematous lung facilitates airway collapse. This means that the expiratory flow drops faster with expiratory volume, the flow-volume diagram becomes concave, and the lung emptying is delayed ( Figs. 11.9A and 11.10 ). Several indices derived from the maximum expiratory flow-volume curve have been suggested for characterization of the types and degrees of flow limitation ( Fig. 11.9B ), among which the volume expired in the first time segment (FEV t ), for example, in the 1st second (FEV 1 ), is the most commonly measured index (see also in the Interpretation and Clinical Application below).



Measurement of Forced Expiratory Flow and Volume in Cooperative Subjects
Summary
- ▪
Spirometry remains the most common lung function test both in children and adults
- ▪
Flow is measured during forced expiration from TLC to RV and a flow-volume curve is constructed
- ▪
Success rate is above 85% in children older than 6 years of age
- ▪
The main outcome measures reported in older children are: forced vital capacity (FVC), FEV 1 , FEV 1 /FVC, forced expired flow between 25% and 75% of expired FVC (FEF 25-75 ).
- ▪
In young children, acceptability criteria differ from those in older children or adults
- ▪
With appropriate training 50%–80% of preschool-aged children are able to perform spirometry although FEV 1 is not the best outcome variable
- ▪
If expiration is long enough, FEV t can be acceptable while forced vital capacity (FVC) and consequently FEF 25–75 and flows at fixed lung volumes may not be valid in preschool-age children
- ▪
Clinical usefulness of the forced expiratory flow and volume variables remains controversial in young individuals with lung disease
Spirometry is the most commonly used lung function method in cooperative children (usually above 6 years of age) and it remains very popular amongst clinicians. The interpretation of the forced expiratory flow-volume curve in children during the teenage years is very similar to that in adults. In the last two decades it has also become evident that children below 6 years of age are able to perform acceptable spirometry after appropriate training. Although forced expiratory maneuvers are feasible, the acceptability criteria and the interpretation of the flow and volume measures are different from those in older children and adults. Later in this chapter, the age-specific limitations, quality criteria, and interpretation of the measures derived from the flow-volume curve will be discussed.
Quality Control, Acceptability Criteria, and Limitations
The criteria for acceptable spirometry are less strict than those in adults; therefore the subjective impression of the technician becomes exceptionally important. The success of the test depends on the effort of the child who has to be continuously instructed and encouraged throughout the maneuvers. If the effort was insufficient, the test is invalid. The “emptying of the lungs” happens much faster in young children than in adults, and the termination of the test differs between individuals. For a valid test, older children and adults are required to exhale at least for 6 s, to accurately determine FVC. This is called the plateau phase of the flow-volume curve, during which the volume is not changing anymore with time. In preschool-aged children a 3-s-long plateau can be accepted; however, young children can usually empty their lungs quicker than 3 s. The technician should be able to recognize whether the child reached RV or finished the expiration too early (so-called premature termination). Premature termination occurs if the expiration is stopped at a flow greater than 10% of the peak flow. Premature termination is the most common reason of unsuccessful test in children, which can be improved with training. In young children FEVt can still be acceptable if they reached RV earlier than 3 s, while FVC should be reported with caution. Artefacts (e.g., cough) are usually recognizable by observation of the flow-volume curve.
The exclusion criteria and the data reporting are fairly consistent in older children, they vary between centers in preschoolers. Three acceptable measurements are desirable; however, the recommendations are flexible on this question. In special cases it should be considered that clinically meaningful information can sometimes be gained from a single successful maneuver. Highest values for FVC and FEV t should be reported, even if they do not belong to the same maneuver. The reproducibility of spirometry in preschool-age children has not been assessed systematically, and this fact contributes to the limited clinical value of spirometry in young individuals (see also the Assessment of Bronchodilator Response below).
Prediction equations for spirometry measures have recently been published in the framework of collaboration between 33 countries (Global Lung Function Initiative), which was established to standardize spirometry worldwide. Age-appropriate lower limits of normal are also available.
Measurement of Forced Expiratory Flow and Volume in Infants
Summary
- ▪
The most common clinical lung function tests during infancy
- ▪
Flow-volume curve can be measured at both FRC (RTC) and in an extended range of lung volumes (RV-RTC)
- ▪
Commercial devices and reference values are available
- ▪
Maximum flow at FRC (RTC) and FVC, FEV 0.4/0.5 , and FEV 0.5 /FVC (RV-RTC) can be reported
- ▪
Flow limitation in the intrathoracic airways can be detected with minimal influence of the nasal pathway
- ▪
Main drawbacks are the sedation and the controversies in the interpretation of the results
Both RTC and RT-RVC were developed for infant use to extend the knowledge of normal lung growth and development, to aid the diagnosis of acute and chronic pulmonary lung disease, and to allow the longitudinal follow-up of lung function from infancy through adulthood. Recommendations and guidelines are available for both techniques (see Suggested Reading ).
Rapid Thoracoabdominal Compression and Raised Volume-Rapid Thoracoabdominal Compression
With RTC, a partial flow-volume curve can be recorded, and the main outcome variable reported is the maximum flow at FRC ( V′ max,FRC , see Fig. 11.10 ). Although this technique had great clinical promise when first introduced, it has been increasingly criticized, especially after the introduction of the RV-RTC. The advantage of the partial flow-volume curve analysis during tidal breathing over RV-RTC is the lack of the unpredictable and variable effects of the lung inflation on the airways.
Infants are usually sedated for the test. They breathe through a pneumotachograph or ultrasonic flow meter that is attached to a facemask. Flow is continuously monitored during the test and volume is calculated by integration of the flow signal. Following the placement of the jacket around the chest and upper abdomen, tidal breathing is recorded for ~30 seconds. Signals of airway-opening pressure, jacket pressure, volume, and flow are displayed real time. A stable EELV is required for at least three consecutive breaths prior to the maneuver; a criterion that is difficult to meet in infants because of their highly variable FRC. Once a stable EELV has been recorded, the jacket is inflated at the end of inspiration with a nonstandardized pressure (30–40 cm H 2 O, different for each device and center) and the flow at the airway opening is recorded during the maneuver. The test is repeated with increasing the inflation pressure stepwise (5 or 10 cm H 2 O) until flow limitation can be demonstrated. The pressure required for the development of flow limitation varies between subjects and it is expected to be significantly lower in children with lung disease (flow limitation develops easier), while in some healthy children pressures as high as 120 cm H 2 O can be insufficient to evoke flow limitation. It also varies between individuals how much pressure is transmitted to the pleural space from the jacket via the chest wall ; therefore comparing the jacket pressure between individuals as the main outcome variable is meaningless. The test finishes when maximum flow has been achieved and no further increase in flow is detected despite the increasing pressure. Similarly to spirometry, the best values of V′ max,FRC are reported instead of the average of all the values. Results are sometimes corrected for lung volume (V′ max,FRC /FRC).
Studies employing an esophageal catheter during RTC have shown that infants can inspire before the end of the maneuver and the chest wall muscle activity can decrease both the intrathoracic pressure and chest wall compliance, resulting in a decreased transpulmonary pressure. It was also shown that if the RTC maneuver was preceded by deep lung inflations, the inspiratory drive of the infant was inhibited. This recognition led to the invention of a new lung function technique: the RV-RTC. This technique is very similar to RTC; however, in RV-RTC the facemask is attached to a T-piece connector (or valves in the modern automatized systems), which contains a valve to separate inspiration and expiration ( Fig. 11.11 ). By occluding the expiratory side of the T-piece, the airway-opening pressure increases until the target pressure (usually 30 cm H 2 O but may differ between centers) is achieved, where activation of the stretch receptors in the lungs results in the relaxation of the respiratory muscles; this is called the Hering-Breuer reflex (see also in the Single Occlusion Technique later in this chapter). At the target pressure the valve opens, which allows the infant to exhale passively. In some centers, cricoid pressure is applied during inflation to prevent the entry of the gas in the stomach. The benefits of this technique, however, have not been addressed systematically and they have been questioned in some studies. The inflation/passive expiration is repeated three to five times and then at the next inflation rapid compression is performed ( Fig. 11.12 ). The variables derived from this maneuver are similar to those measured with spirometry in cooperative subjects. In most of the centers where RV-RTC is performed routinely, the measurement of partial flow-volume curve is undertaken first to record V′ max,FRC (the measurement of V′ max,FRC with RV-RTC is not reliable as the lung inflations can change EELV) and to establish the ideal jacket pressure for the rapid compression in RV-RTC.


Quality Control, Acceptability Criteria, and Limitations
Once the infant is ready for the lung function test (either RTC or RV-RTC), a jacket matching the infant’s size should be selected carefully. The infant is then wrapped in the jacket to cover as much of the chest and the upper abdomen as possible; however, the jacket should not interfere with the breathing of the baby. If the jacket pressure is other than zero, or if it is changing during tidal breathing, then the jacket is too tight.
Following the 30-second tidal breathing recording, a short occlusion should be applied to check the system for leaks. If there is a drop in the airway-opening pressure during the occlusion it indicates an insufficient seal around the facemask, while if flow is detected in the system it suggests a leak through the occlusion valve (or T-piece in RV-RTC). As in other lung function tests, the correct placement of the mask is crucial. Leaks are more frequently present during the inflation phase of RV-RTC when the airway-opening pressure increases. In contrast, pressing the facemask too hard might result in the occlusion of the upper airways (and underestimation of the measured flow).
Artefacts are frequently present during the test. The compression-decompression of the chest might activate muscle and respiratory reflexes, resulting in muscle constriction and sometimes glottic closure during the test. The latter is easily recognizable by the observation of the flow-volume curve. Respiratory reflexes (e.g., early inspiration) can also interfere with the measurements. Negative effort dependence, with decreasing expiratory flows and a concave flow-volume curve, can be seen if the jacket pressure is too high, leading to external compression of the chest wall and large intrathoracic airways.
Normative values are available for both techniques, and device-specific reference values have been published recently for RV-RTC ( Fig. 11.13 ). Z -scores have also been calculated for a mainly Caucasian population. If z -scores are not relevant for the measured population, absolute values should be reported instead of the percent predicted values. Values of forced expiratory flows and volumes measured with RTC and RV-RTC show a markedly nonlinear relationship with body length and weight and a linear relationship with age during infancy. Interestingly, forced expiratory flows are generally higher in girls than boys.

If multiple lung function tests are performed in the same infant, RTC or RV-RTC should be performed as the last tests, because these radical pressure changes may influence the baseline respiratory mechanics and measurements of ventilation inhomogeneity. It has recently been published that the inflation/compression maneuvers necessary for RV-RTC affect the value of lung clearance index (LCI) measured with MBW (LCI is lower after the maneuver). However, if the most relevant clinical (or research) question is related to the forced expiratory variables, it always has to be considered that the time of the proper sedation (30–45 minutes) might not be sufficient to undertake multiple lung function tests. In this case (RV-) RTC may need to be prioritized.
Rapid Thoracoabdominal Compression-Specific Considerations
Once acceptable flow-volume curves are detected, the best value for V′ max,FRC should be reported and it is desirable if it is within 10% from the second highest value. From the three best measurements the coefficient of variation (COV: 100[SD/mean]) can be calculated. The values of COV are usually higher than 10%, which is likely to be explained by the changes in the absolute lung volume between measurements (even if EELV was reproducible within one test).
Raised Volume-Rapid Thoracoabdominal Compression-Specific Considerations
The jacket pressure has to be selected carefully, since if the jacket pressure is not high enough, it will decrease the value of FEV t (while it may not affect FVC); if the jacket pressure is too high, it can cause airway obstruction.
The test is technically acceptable if the relaxation is complete and it lasts until RV is reached. This is easily recognizable by visual observation of the flow-volume curves. A test meets the quality criteria if it is artifact-free (no closure, no inspiratory effort, etc.) and if the peak flow is achieved before 10% of the volume is expired. At least two technically acceptable maneuvers with FVC (and FEV t ) within 10% are required for a meaningful interpretation. Forced expiratory volumes and flows are reported from the best maneuver.
Interpretation and Clinical Application of the Flow-Volume Curve
Independently of the patient’s age and the technique used to assess forced expiratory flows or volumes, the flow-volume and the volume-time curves should always be observed carefully. This is crucial for the correct interpretation of the test. The interpreter should be able to recognize if the maneuver was not performed correctly, be aware of the presence of artefacts, and—if the examiner is experienced enough—the shape of the curve can provide unique clinical information ( Fig. 11.9A ).
While V′ max,FRC is the most commonly reported measure of the RTC, various outcome variables have been obtained from RV-RTC. The relevance of FEV 1 in infants and preschool-aged children has been questioned because of the anatomical and physiological differences in the respiratory system between infants and preschool-aged children and adults. In infants, the airways are relatively big compared to the lung volumes; therefore the emptying during a forced expiration happens much faster (the lungs are basically “empty” after the first second). Therefore the most commonly reported variable is the FEV 0.5 , which reflects the expired volume over the first 0.5 seconds of a forced expiration. In preterm infants and neonates, FEV 0.4 has also been introduced. In preschool-age children it is still undecided which variable should be reported from the flow-volume curve. Although FEV 1 has been commonly used in both clinical practice and research studies, FEV 1 has proven insensitive for airway obstruction in children below 6 years of age. As a consequence, reporting the FEV values either for 0.5 or 0.75 seconds has become more popular over the last years. In older children, FEV 1 is the most commonly reported measure of lung function and has been used worldwide to aid the diagnosis or to track the progress of lung disease. Healthy subjects are able to empty about 80%–90% of their FVC in the 1st second of a forced expiration. In the presence of airway obstruction, flow limitation appears at higher lung volume delaying expiration. A normal or increased FVC with a decreased FEV 1 and hence an FEV 1 /FVC ratio below 80% (or −1.64 z-score) suggests airway obstruction. In contrast, diminished FVC and FEV 1 with a normal (i.e., >80%) FEV 1 /FVC ratio usually reflect restrictive lung disease (if the forced expiratory maneuver was performed correctly). FEV 1 /FVC has been reported as a more sensitive indicator of early lung disease than FEV 1 alone. The ratio of FEV t to FVC as a measure of airway obstruction offers very limited clinical potential in children below 6 years of age. As discussed above, this is most probably due to the anatomical and physiological differences in the respiratory system between children and adults and hence an invalid FVC. It is important to note that the FEV t values, whichever t is selected for the analysis, cannot be directly compared between infants and older children. Additionally, when longitudinal measurements of forced expiratory flow are interpreted, it has to be considered that infants were measured during sedation in the supine position and the measurements were obtained through a facemask.
From the measures of the forced expiry flow, FEF 25-75 was suggested as a measure of small airway function and a sensitive marker of early disease. This value is read as the slope of the line connecting the points between 25% and 75% of the expired FVC and it represents the mid-portion of forced expiratory maneuver. This site of the flow limitation is assumed to reflect small airway obstruction; however, the usefulness of FEF 25-75 is still under debate especially in children. It is highly variable in children, and a recent study reported that FEV 1 /FVC was more sensitive to detect early disease in both CF and asthma in children above 6 years of age. The other popular measure of flow during forced expiration is the maximum or peak expiratory flow (PEF). This can even be measured with a peak flow meter where the child is instructed to take the biggest possible inspiration and blow out as quick and hard as they can. Variabilty in PEF was suggested as an indicator of poor asthma control and hence this test is still frequently included in the clinical management of children. However, it is still not well-established how much this information adds into the clinical management of an individual. The device is cheap and once the child learns the technique it is easy and quick to perform daily even at home. The best of 3 or 5 attempts should be recorded.
In children with cystic fibrosis (CF) the clinical application of spirometry is controversial. It has been shown that structural lung disease begins in early life and can be seen on a chest computed tomography (CT) scan in preschool- and school-aged children with CF while their spirometry results are still normal. Despite this fact, spirometry is used in most studies of CF, and forced expiratory flow and volume measures are still the main outcome measures in clinical trials. It has been recognized in recent years that other lung function tests (e.g., MBW) might be more useful in detecting abnormalities of the respiratory system in early CF. Despite these controversies, spirometry is considered the standard test for monitoring lung disease in CF to detect changes in lung function or response to treatment. FEV 1 is traditionally included in the evaluation protocols for lung transplantations in CF-related terminal lung disease: an FEV 1 value below 30% predicted is one of the indications to be placed on a lung transplant waiting list. Following lung transplantation, repeated spirometry is the only lung function test that is routinely performed to diagnose chronic allograft dysfunction, even if it does not seem to be the most sensitive test to detect bronchiolitis obliterans. In contrast, the RV-RTC method has provided meaningful clinical information on flow limitation in infants with CF. Since newborn screening of CF is routinely performed in many countries worldwide, the need for a sensitive lung function test that detects early lung disease, does not require sedation, and can be undertaken easily and safely in young children is high.
In young wheezy and asthmatic children, significantly lower values of FEV 0.5 and FEV 0.75 have been reported compared to those in healthy children; however, it is unclear how informative the spirometry is in the clinical management of an individual. It is likely that the test is more useful in “older” preschoolers (i.e., 5 and 6 years of age) and school-age children, and their longitudinal follow-up with spirometry provides important information on the progression of their lung disease. The correct interpretation of abnormal lung function in older children can confirm the diagnosis of asthma. These indices include (1) a low FEV 1 (below −1.64 z-score), (2) a low FEV 1 /FVC (below −1.64 z-score), and (3) an exaggerated variability in PEF (morning-to-evening more than 20%). Children do not always have airflow limitation on the day of the test and their lung function is often normal when measured with the spirometry between two exacerbations. Therefore it is worthwhile to perform BDR or bronchoprovocation test: a positive BDR (see below) or a confirmed bronchial hyperresponsiveness (see Chapter 12 ) can support the diagnosis of asthma. It has recently been suggested that different obstruction phenotypes defined with spirometry are related to different risk of poor asthma outcomes such as exacerbation or medication use in children. In poorly controlled or severe asthma, deep inspiration and forced expiration per se can induce bronchospasm and worsen lung function. This is rarely seen in children and when it is present it is difficult to produce reproducible flow-volume curves. Decreasing FEV 1 with each maneuver can be a sign of severe asthma and should be interpreted accordingly. It is important to note here that spirometry can be very difficult and tiring to perform for children with severe lung disease and alternative tests should be considered. Interestingly, it has been suggested that variables derived from the forced expiratory flow-volume curve during infancy (both during tidal breathing and following lung inflation) not only discriminate between health and disease, but also that the early presence of flow limitation predicts lung disease later in life Attempts have also been made to assess bronchial hyperresponsiveness in wheezy infants ; however, it remains unclear whether it adds any value to the clinical management of individuals.
In research studies, variables derived from the spirometry are the most commonly used outcome measures in clinical trials, studies on lung growth and development, and classification of wheezy and asthmatic children.
The Assessment of Bronchodilator Response
The assessment of the response to albuterol is one of the most frequently performed tests in both clinical practice and research studies. The main limitation of the measurement of bronchodilator response (BDR) in infants and preschool-age children is the high intrasubject variability of the forced expiratory flow-volume curves, which makes the characterization of the bronchodilator-related changes in lung function difficult. Since the forced flow-volume curve is highly reproducible in older children, this problem is far less significant.
In infants, the therapeutic use of albuterol was questioned for a long time because of the lack of objective and sensitive lung function techniques in early life. Since RTC is able to detect flow limitation, it had great promise in the assessment of diagnostic or therapeutic drug interventions. Unfortunately, these tests have revealed the most important clinical limitation of the test: the uncontrollable changes in the absolute lung volume following an intervention. A stable EELV is required for the proper interpretation of the results; however, this is difficult to achieve in infants. Although EELV equals FRC (where the lung and chest wall recoils are equal but opposite in sign, also called equilibrium volume) in a quietly breathing adult, this assumption is not always fulfilled in infants, especially in the presence of lung disease. Therefore EELV may change after the administration of albuterol and the flow-volume curves are obtained on different absolute lung volumes before and after albuterol. Since flow is measured at “FRC,” it may appear—misleadingly—that the bronchodilator treatment had no beneficial effect (or even had an inverse effect) ; however, most probably only the lung volume changed and consequently the maximum flow at FRC decreased. This is a well-documented limitation of the RTC and explains the controversies in the interpretation of BDR when measured with this technique.
Flow-volume curves obtained with the RV-RTC have been shown to be more reproducible and hence more useful in the detection of BDR ( Fig. 11.14 ). When the two techniques were compared in the same infants, no changes were detected with RTC in children with acute viral bronchiolitis, while more than half of the children had a positive response to albuterol when assessed with RV-RTC. When changes in individuals were analyzed, V′ max,FRC was unchanged or decreased in all of the subjects. The fact that previous studies have reported both positive and negative BDR during infancy precluded the establishment of the optimal response to albuterol that differentiates health and disease with the highest specificity and sensitivity for any of the variables.

Positive BDR as assessed with the spirometry is usually defined as ≥12% and/or 200 mL improvement in FEV 1 in older children, but this is not appropriate in children younger than 6 years of age. A positive BDR assessed when the child is symptom-free can strengthen the diagnosis of asthma. On the basis of the limited data available in preschool-age children on BDR and spirometry, FEV 0.75 has been suggested to have the biggest potential to detect positive BDR in children between 3 and 6 years of age. In a relatively small population of 43 asthmatic and 22 healthy children, 14% improvement in FEV 0.75 was defined as a positive response. A more recent study on a much larger population of healthy ( n = 431) and asthmatic ( n = 289) children has suggested that 11% increase in FEV 0.75 was 51% sensitive and 88% specific to detect altered lung function in asthmatic children.
Measurement of Resistance and Compliance
Resistance and compliance are the most important mechanical properties of the respiratory system. The measurement of respiratory resistance is the most frequently chosen alternative lung function test in clinical practice in preschool-age children who are not able to do spirometry. The resistance of the airways is highly dependent on the airway caliber; therefore the measurement of resistance can be informative in both obstructive and restrictive lung diseases. Resistance describes the relationship between pressure (P) and flow (V′), and combines the determinants of energy dissipation in the airways and the respiratory tissues. Compliance describes the relationship between P and volume (V), and characterizes the ability of the tissues to expand in response to distending pressure and to store elastic energy. Both resistance and compliance depend on (1) the range of deformation of the respiratory system and (2) the rate (frequency) of the deformation. These factors make the comparison of measurements with different techniques difficult.
Several techniques are available for the measurement of resistance and compliance in infants and preschool-age children both during dynamic and quasi-static conditions. Although the techniques differ in their measurement principles and outcome measures, the feasibility in different patient groups, and the level of cooperation required, the main physiological assumptions of the measurement and interpretation of resistance and compliance apply to all of these techniques; therefore they will be discussed together below.
Physiological Principles and Assumptions
Breathing is accomplished by the rhythmic action of the respiratory muscles, which create forces to overcome the opposing elastic and viscous forces of the lungs and also other structures involved in the respiratory movements, including the muscles themselves. Knowledge on the mechanical properties of the lungs and chest wall is important not only for the assessment of the work needed to maintain breathing but also because significant alterations in elastance and resistance may occur as a result of pathological processes. In infancy and childhood, it is also important to know whether the values of mechanical parameters observed in a subject are in accordance with those predicted for the particular stage of lung development.
The respiratory muscles are responsible for the tidal expansion of a passive system (i.e., lungs and upper airways), and therefore they have to overcome a pressure (the so-called transpulmonary pressure, P tp ) that arises from the elastic, resistive, and inertial forces of the lungs during tidal breathing. In general, we can describe this pressure as
P tp = P 0 , L + P el , L + P res , L + P i , L
P tp = P 0 , L + E L × V + R L × V ′ + I L × V ″ ,
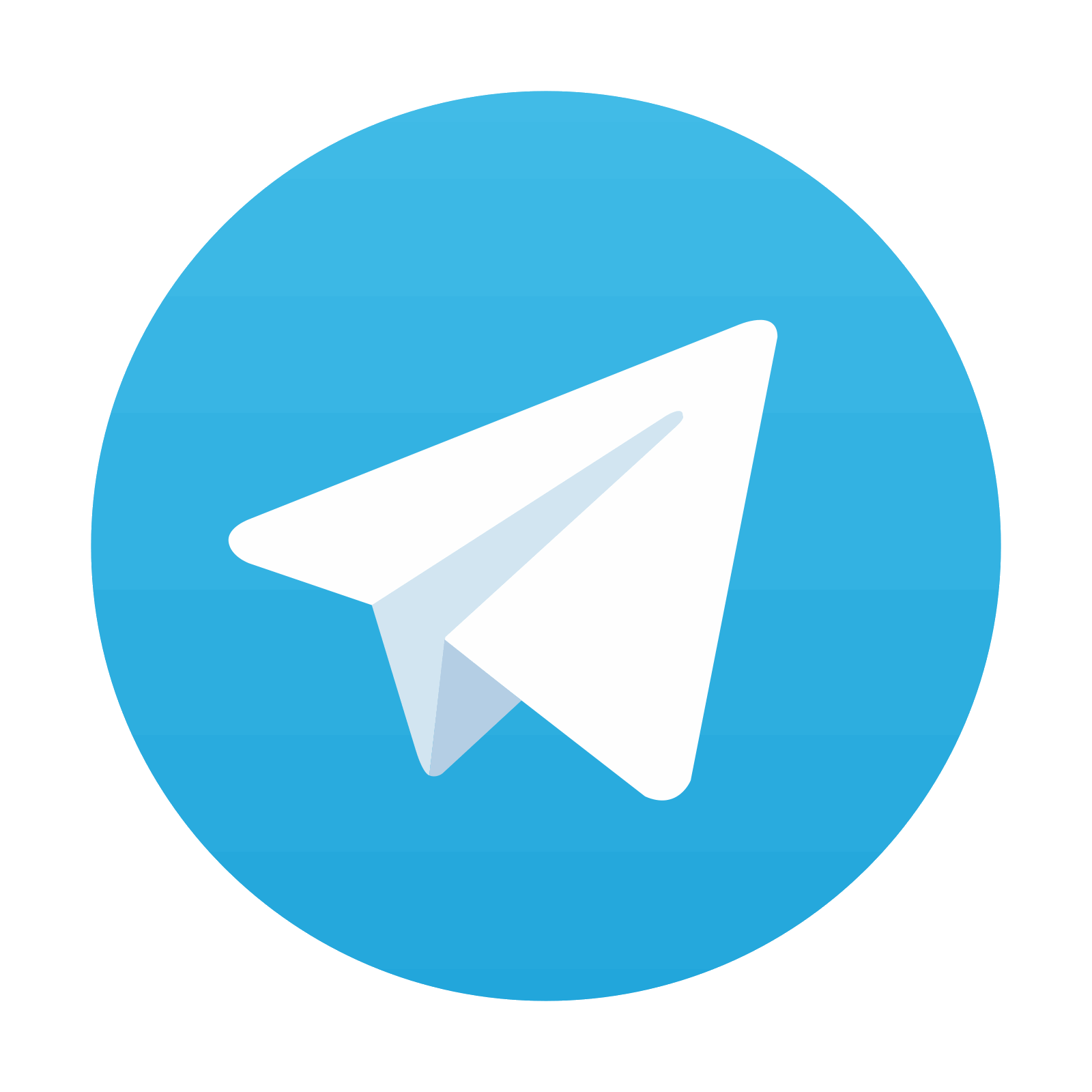
Stay updated, free articles. Join our Telegram channel
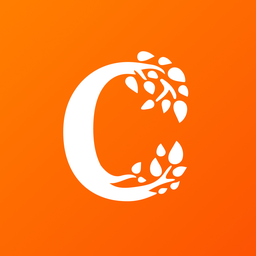
Full access? Get Clinical Tree
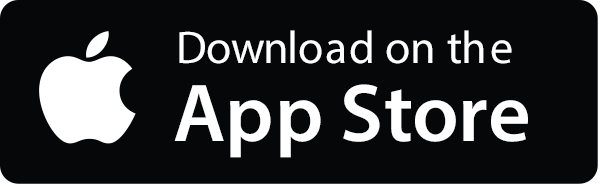
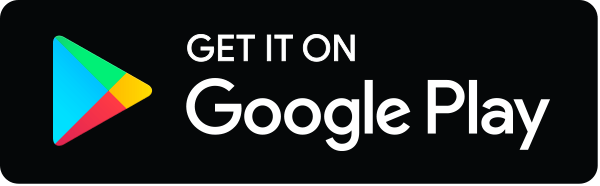