Background
Echocardiography is a valuable noninvasive technique to estimate cardiac output (CO) from the left ventricle (LV) not only in clinical practice but also in small-animal experiments. CO is used to grade cardiac function and is especially important when investigating cardiac injury (e.g., myocardial infarction [MI]). Critically, MI deforms the LV, invalidating the assumptions fundamental to calculating of cardiac volumes directly from the LV. Thus, the purpose of this study was to determine if Doppler-derived blood flow through the pulmonary trunk (pulmonary flow [PF]) was an improved method over conventional LV–dependent echocardiography to accurately determine CO after MI.
Methods
Variations in CO were induced either by transverse aortic constriction or MI. Echocardiography was performed in healthy ( n = 27), transverse aortic constriction ( n = 25), and MI ( n = 41) mice. CO calculated from PF (pulsed-wave Doppler) was internally compared with CO calculated from left ventricular images using M-mode (Teichholz formula) and the single-plane ellipsoid two-dimensional (2D) formula and externally compared with the gold standard, flow probe CO.
Results
In healthy mice, all three echocardiographic methods (M-mode, 2D, and PF) correlated well with flow probe–derived CO. In MI mice, only PF CO values correlated well with flow probe values. Bland-Altman analysis confirmed that PF was improved over M-mode and 2D echocardiography. Inter- and intrauser variability of PF CO was reduced, and both inter- and intraclass correlation coefficients were improved compared with either M-mode or 2D CO calculations.
Conclusions
PF CO calculated from pulsed-wave Doppler through the pulmonary trunk was an improved method of estimating CO over LV–dependent formulas after MI.
Highlights
- •
Left ventricular infarction drastically alters chamber shape and wall kinetics.
- •
This invalidates assumptions for calculating cardiac output (CO).
- •
Pulmonary flow (PF) precisely and accurately determines CO.
- •
PF is easily determined in experimental models of heart failure.
- •
PF reduces user bias and variability in determining CO.
Cardiac output (CO), the product of heart rate and stroke volume, is an important measure of cardiac performance. Although various methods exist for estimating CO (e.g., thermodilution, pulse pressure, magnetic resonance imaging, etc.) these techniques are generally invasive, are impractical in experimental models, or require highly specialized and expensive equipment. Both clinically and experimentally, echocardiography remains a frequently used method to rapidly and noninvasively estimate CO with low overhead cost for equipment and training.
The ability to accurately determine CO is critical for assessing and comparing myocardial function. Using echocardiography to determine CO is commonly accomplished by calculating left ventricular (LV) volumes directly from one-dimensional (1D) and two-dimensional (2D) images of the LV. Yet these calculations are limited when the shape of the LV no longer adheres to the assumptions defined by the formulas. Two common formulas are the 1D “M-mode” formula described by Teichholz et al . and the 2D single-plane ellipsoid formula. These formulas were derived under the assumption that the LV resembles an approximated “ellipsoid” shape with uniform wall movement through each plane (vertical plane; M-mode, long-axis plane; 2D). The accuracy with which these 1D and 2D methods estimate stroke volume (diastolic volume − systolic volume) is thus contingent on the LV’s maintaining both this ellipsoid shape and uniformity of contraction.
Directly determining CO using these formulas is robust and comparable in models without perturbations in cardiac symmetry—healthy or hypertensive hearts (e.g., transverse aortic constriction [TAC])—in which the LV is symmetric, approximates an ellipsoid shape, and exhibits uniform contraction. These formulas are fallible when the LV no longer adheres to these assumptions, as is the case after myocardial infarction (MI) (see Figure 1 ). Here, (1) the chamber is dilated and the LV shape distorted, (2) the LV wall is nonuniform with both hyper- and hypocontractile myocardial tissue and/or akinetic scar, and (3) pathology interferes with proper landmarking (e.g., nonvisible papillary muscles). Although these limitations are recognized, and although caution is suggested when using 1D and 2D approximations of LV volumes in ischemic models (e.g., MI), LV–dependent echocardiographic methods for determining CO continue to be relied on. Accordingly, the purpose of this study was to identify an improved echocardiographic method to grade CO after MI that is more precise and accurate.

In large-animal MI models (e.g., porcine, ovine, and canine), the complications associated with single-image formulas can be avoided by calculating the volume of multiple cross-sectional images, collectively accounting for differences in chamber shape and contractile function between different ventricular segments. In small-animal models of MI (e.g., murine, cricetine, and leporine), chamber size, heart rate, and poor resolution of the thinly ballooned scar impair the ability to obtain multiple cross-sectional volumes with reproducible accuracy. Indeed, an improved method would determine CO regardless of LV pathology. In 2011, Tournoux et al . introduced the use of pulsed-wave Doppler blood flow through the pulmonary trunk (termed pulmonary flow, PF) as an alternative method to approximate CO in healthy mice and following acute endotoxic shock. What has yet to be investigated is whether this methodology can be applied to the most common experimental models of heart failure, for example, pressure overload (i.e., TAC) or volume overload (i.e., MI). In this study, we present data establishing PF as a preferable method over classical LV–dependent echocardiographic formulas for calculating CO, particularly in a mouse MI model, both increasing the accuracy and reducing the variability of CO measurements. This has relevance when investigating treatments for MI.
Methods
Surgical Model
Briefly, 8- to 9-week-old male CD-1 mice (∼35 g body weight) were anesthetized with isoflurane/oxygen (2%:100%), intubated, and ventilated (Harvard Apparatus, Holliston, MA) at 150 breaths/min at a tidal volume of about 300 μL. Animals were randomly assigned to receive either MI or TAC. In the MI group, a thoracotomy was performed on the left side of each animal to expose the left anterior descending coronary artery, which was ligated with 7-0 Surgipro II polypropylene suture (Covidien, Dublin, Ireland) directly inferior to the left atrium. MI was confirmed by myocardial blanching. In the TAC group, the cartilaginous connection between the sternum and ribs 2 and 3 was separated to expose the upper mediastinum. The transverse aorta was isolated and subsequently set with 7-0 Sofsilk thread (Covidien) to the diameter of a blunted 26-gauge needle. For both surgical models, the ribs and skin were closed using 5-0 Sofsilk suture. Mice were taken off anesthetic and allowed to recover on 100% oxygen. Housing and experimental procedures were approved by the animal care committee at the University of Guelph and were in accordance with the guidelines for laboratory animal welfare set forth by the University of Guelph.
Echocardiographic Analysis
Images were obtained between 9 am and 3 pm using the Vevo2100 system (VisualSonics, Toronto, ON, Canada). All TAC mice were evaluated 18 weeks after surgery. All MI mice were evaluated 4 to 8 weeks after surgery. Mice were anesthetized with isoflurane/oxygen (1%–1.5%:100%) just below the level of a pedal reflex to ensure comparable anesthesia and maintained at 37°C throughout procedures. All LV images were acquired within 10 min of induction with the MS550D ultrasound transducer set to 40 MHz. All measurements were done using the Cardiac Package (VisualSonics). M-mode (1D) images were analyzed using the LV-trace function, and long-axis B-mode (2D) images were analyzed using the 2D area calculation. M-mode images were obtained from either long- or short-axis views of the heart at the midpapillary level. Two-dimensional images were acquired from the long-axis view of the LV, where probe placement was such that the LV chamber was visualized from the apex to the outflow tract.
PF was measured from a parasternal short-axis view of the pulmonary trunk just distal of the pulmonary semilunar valves with probe placement arranged to obtain an image as depicted in Figure 2 D, similar to the long-axis view of the LV but with the probe moved 1 to 2 mm superiorly. Aortic flow was measured from a parasternal long-axis view of the ascending aorta ( Supplemental Figure 1 , available at www.onlinejase.com ). Pulmonary and aortic flows were calculated as the product of the vessel area (2π r 2 ) and the velocity-time integral (VTI) of the pulsed-wave Doppler of flow at that level ( Figure 2 C, Supplemental Figure 1 D, available at www.onlinejase.com ). Vessel diameter was measured just distal to the valve leaflets where VTI was obtained. Doppler gain was consistently set between 32 and 35 dB at a frequency of 32 MHz to limit color Doppler variability. Beam steering, a novel function of the Vevo2100 system, was used to ensure that the 2D angle between the direction of the pulmonary trunk visualized in vivo and the sound beam(s) was <45°.

All echocardiographic images (M-mode, 2D, and flows) were obtained in triplicate. All volumes were calculated as the mean of three images sets; the mean of each set was five consecutive heartbeats. All images were reanalyzed in a blinded manner to identify any subjectivity, which was defined as any mean exceeding 10% between repeated measures. In all cases of discrepancy, image quality was poor in part or in entirety, and values were excluded from the analysis (see Table 1 ). All images were acquired within 10 min of induction, and calculations were done after acquisition.
PF (pulmonary trunk) | M-mode (left ventricle) | 2D (left ventricle) | Aortic flow (ascending aorta) | Flow probe (aorta) † | 3D MRI (left ventricle) | Thermodilution | |
---|---|---|---|---|---|---|---|
Used in this study | Yes | Yes | Yes | Yes | Yes | No | No |
Frequency of quality images ∗ | Control = 100% MI = 95% TAC = 92% | Control = 96% MI = 90% TAC = 92% | Control = 92% MI = 84% TAC = 73% | Control = 73% MI = 75% TAC = N/A | Control = 93% † MI = 93% † TAC = N/A | NA | NA |
Reproducibility of landmarks for image acquisition | Control = ✓ MI = ✓ TAC = ✓ | Control = ✓ MI = ╳ TAC = ✓ | Control = ✓ MI = ╳ TAC = ✓ | Control = ✓ MI = ✓ TAC = ╳ | Control = ✓ MI = ✓ TAC = ✓ | NA | NA |
Measures forward flow | ✓ | ╳ | ╳ | ✓ | ✓ | ╳ | ✓ |
Independent of LV wall composition/kinetics | ✓ | ╳ | ╳ | ✓ | ✓ | ✓ | ✓ |
Light anesthesia | ✓ | ✓ | ✓ | ✓ | ╳ | ╳ | ╳ |
Noninvasive | ✓ | ✓ | ✓ | ✓ | ╳ | ✓ | ╳ |
Spontaneous breathing | ✓ | ✓ | ✓ | ✓ | ╳ | ✓ | ╳ |
Estimated time to acquire CO (total anesthetic time) (min) | 5–10 | 5–10 | 5–10 | 5–10 | 15–30 | 15–30 | ∼30 |
∗ The image exclusion criterion was an intrauser-calculated CO varying by >10% upon blinded re-analysis.
† Invasive techniques present an increased risk for losing data to surgical complications.
Flow Probe
Similar to surgical intervention, mice were intubated and ventilated (Harvard Apparatus) at 150 breaths/min at a tidal volume of about 300 μL with an isoflurane/oxygen mix (2%:100%). Mice were maintained at 37°C with a rectal probe and heating lamp throughout data collection. Ribs were separated at the cartilaginous connections of ribs 2, 3, and 4 to expose the upper mediastinum. A piece of 7-0 silk thread was looped around the ascending aorta used and to delicately place the aorta inside the 1.5-mm-diameter flow probe (Transonic, Ithaca, NY). This flow probe was connected to the TS420 Perivascular Flow Module, and CO calculations were presented as a digitized readout directly on the machine. Probe placement was adjusted to obtain peak flow, and CO was recorded when peak flow values were maintained for at about 3 consecutive seconds (approximately 30 heartbeats). In contrast to previous studies, flow probe CO calculations were made 24 hours after echocardiographic CO calculations, as flow probe placement directly impeded concurrent echocardiographic visualization of the pulmonary trunk.
CO Calculations
The following formulas were used to calculate cardiac volumes from echocardiographic images (in all cases, CO was the product of stroke volume and heart rate):
M-mode (1D): volume = [7.0/(2.4 + D )] D 3 , where D is the internal “diameter” at the midpapillary region of long-axis B-mode images in either systole or diastole. Stroke volume was calculated as the difference between diastolic and systolic volumes.
2D: volume = [(8 × A 2 )/(3π L )], where A is longitudinal surface area, and L is chamber length. 2 Stroke volume was calculated as the difference between diastolic and systolic volumes.
PF/aortic flow: volume = (VTI)(2π r 2 ). Flow-derived stroke volumes were calculated as a product of the VTI and the vessel cross-sectional area (pulmonary trunk or ascending aorta) at the point of peak flow, where r is vessel radius.
Variability Assessments
Variability of echocardiography-derived CO values (M-mode, 2D, and PF) was assessed within users (intrauser variability) and between users (interuser variability) and is summarized in Table 2 . A total of nine images, three with each method, were collected per animal ( n = 11 for healthy mice, n = 9 for MI mice). All images were acquired rapidly over a period of <10 min to help ensure similar heart rates and body temperatures. CO from each image was averaged over five heartbeats (between breathes). Intrauser variability was calculated from the SD within each set of three images (M-mode, 2D, or PF) and expressed as a percentage of the average CO for that method in that particular mouse. The intraimage variability per user was averaged per mouse ( n = 3) and the reported intrauser percentage is the average variability between the two users. Interuser variability was calculated as the average absolute percentage difference between user 1’s and user 2’s calculations from the same images ( n = 30–36 per method). Intra- and interuser reliabilities were assessed using intra- and interclass correlation coefficients respectively, and values are presented in Table 3 . Correlation coefficients were calculated in Excel (Microsoft, Redmond, WA) using the “Anova: Two Factor without Replication” data analysis table (i.e., a repeated-measures analysis) and the formula {[MSRow − MSE]/[MSRow + (dfCol)(MSE) + (dfCol + 1)(MSCol − MSE)/(dfRow + 1)]} (adapted from Weir ).
∗ Significance from PF as determined by one-way analysis of variance, P < .05.
Intraclass correlation coefficient | Interclass correlation coefficient | Intraclass correlation coefficient | Interclass correlation coefficient | ||
---|---|---|---|---|---|
Healthy | MI | ||||
M-mode | 0.902 | 0.679 | M-mode | 0.519 | 0.119 |
2D | 0.834 | 0.692 | 2D | 0.625 | 0.351 |
PF | 0.881 | 0.833 | PF | 0.817 | 0.754 |
Histologic Approximation of Infarct Size
A total of 10 mice were blindly selected for infarct size estimation following data collection. Briefly, hearts were perfusion-fixed with 1 × PBS, 50 mmol potassium chloride and 10% neutral buffered formalin (VWR International, Mississauga, ON, Canada) through the right common carotid artery. Hearts were processed overnight and embedded in paraffin. Cross-sectional slices (5 μm) were obtained from the approximated apical, midpapillary, and basal regions of the heart. Sections were stained with Gomori’s One Step Trichrome (Poly Scientific, Bay Shore, NY). Images were acquired using an Olympus FSX 100 light microscope and analyzed using Cell Sense software (Olympus, Tokyo, Japan). Infarct size was determined as a percentage of total LV circumference as averaged from all three sections. Average infarct size using this method was 55.2 ± 9.7% of the total LV circumference.
Bland-Altman Analysis
Bland-Altman graphs were created in Prism 6.0 (GraphPad Software, La Jolla, CA) to evaluate the agreement between reference values (e.g., flow probe) and comparator values (e.g., M-mode, echocardiography). Zero-bias lines are presented as the “ideal agreement” between the two compared methods across a range of differences. Regression lines are plotted to demonstrate the directional biases, that is, whether a bias is more likely to exist above or below a particular inflection point. The difference between the reference and comparator values was plotted against the average of these values to reduce the risk for biasing trends. Mean difference, 95% CI, and range are also presented.
Statistical Analysis
Averages are presented as ±SEM unless otherwise indicated. SD, Pearson correlation, and Bland-Altman (Tukey mean difference) plot analysis with an absolute mean/range difference were determined using Prism 6.0. All other variables were compared using either Student’s t test (for paired values) or one-way analysis of variance for group comparisons; P values < .05 were considered to indicate statistical significance in all cases. Because higher n values increase absolute inter- and intraclass correlation coefficient values, we compared a similar number of CO values for the these calculations ( n range = 32–36). inter- and intraclass correlation coefficient values were then compared against one another to determine relative reliability of each technique.
Results
We compared three methods of echocardiography-derived CO (M-mode, 2D, and PF) to a literature gold standard for CO (flow probe) in healthy mice and two experimental models of heart failure (i.e., TAC and MI). Complete echocardiographic values are summarized in Table 4 .
Healthy | TAC | MI | |
---|---|---|---|
M-mode | n = 26 | n = 23 | n = 37 |
EDD (mm) | 4.74 ± 0.05 | 5.16 ± 0.13 ∗ | 6.29 ± 0.12 ∗ |
ESD (mm) | 3.37 ± 0.08 | 4.46 ± 0.20 ∗ | 5.83 ± 0.15 ∗ |
EF (%) | 55.5 ± 1.4 | 29.4 ± 3.4 ∗ | 16.7 ± 9.6 ∗ |
FS (%) | 29.1 ± 0.9 | 14.3 ± 1.8 ∗ | 7.8 ± 0.8 ∗ |
SV (μL) | 59.1 ± 1.4 | 33.1 ± 2.7 ∗ | 30.0 ± 2.1 ∗ |
HR (beats/min) | 533 ± 9 | 572 ± 10 ∗ | 553 ± 8 ∗ |
CO (mL/min) | 31.4 ± 1.0 | 18.9 ± 1.5 ∗ | 16.6 ± 1.1 ∗ |
2D (long-axis) | n = 23 | n = 19 | n = 27 |
---|---|---|---|
Diastolic length (mm) | 7.47 ± 0.16 | 8.88 ± 0.24 ∗ | 9.24 ± 0.15 ∗ |
Systolic length (mm) | 6.51 ± 0.15 | 8.21 ± 0.17 ∗ | 8.71 ± 0.18 ∗ |
Diastolic area (mm 2 ) | 31.0 ± 0.6 | 38.9 ± 2.0 ∗ | 53.00 ± 2.20 ∗ |
Systolic area (mm 2 ) | 19.4 ± 0.7 | 33.2 ± 1.5 ∗ | 48.10 ± 2.52 ∗ |
EF (%) | 55.3 ± 1.4 | 24.1 ± 3.0 ∗ | 15.2 ± 1.6 ∗ |
FAC (%) | 37.7 ± 1.0 | 16.6 ± 2.2 ∗ | 10.1 ± 1.0 ∗ |
SV (μL) | 55.9 ± 1.2 | 30.0 ± 2.5 ∗ | 31.8 ± 2.5 ∗ |
HR (beats/min) | 535 ± 8 | 580 ± 12 ∗ | 548 ± 14 |
CO (mL/min) | 29.6 ± 0.6 | 17.4 ± 1.5 ∗ | 17.3 ± 1.5 ∗ |
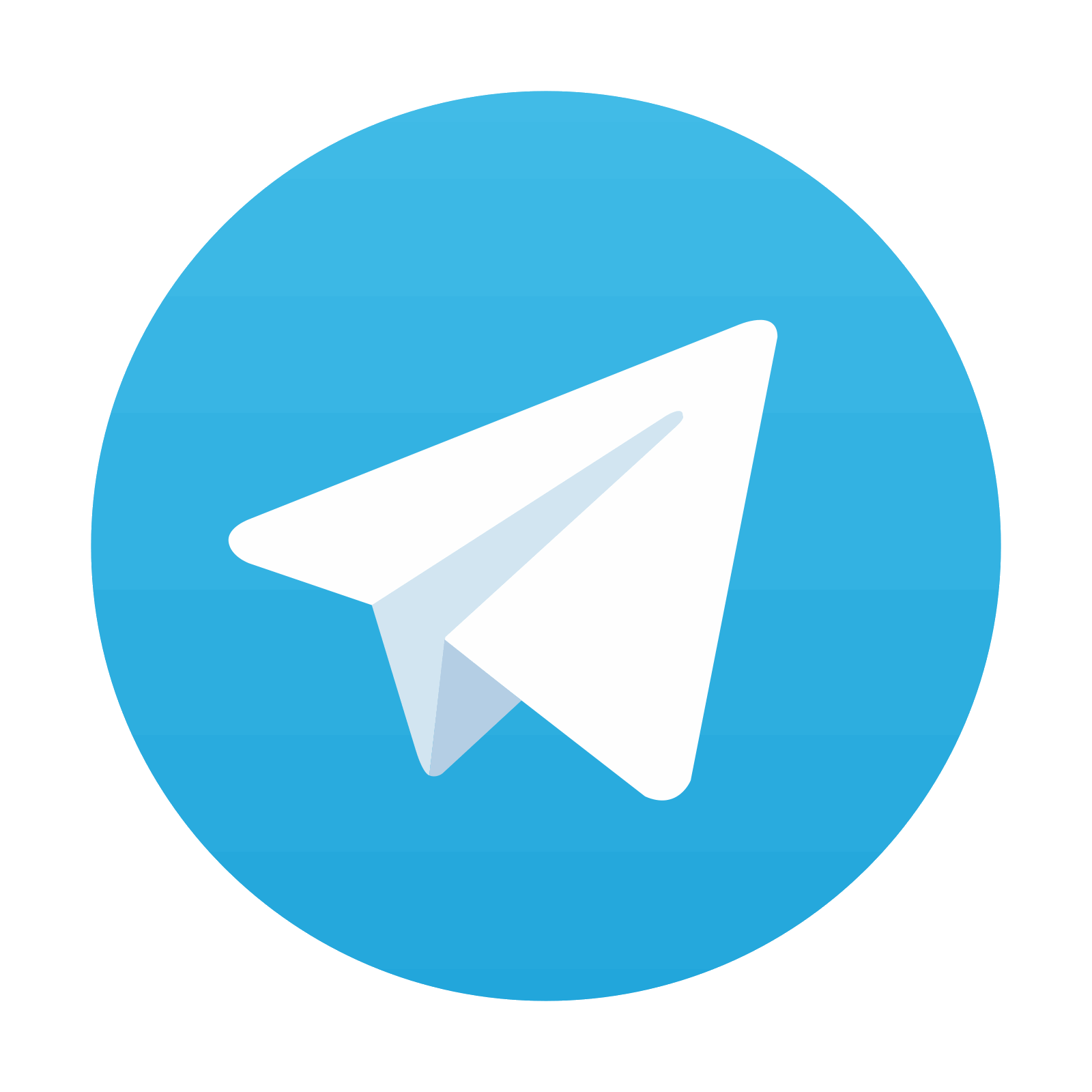
Stay updated, free articles. Join our Telegram channel
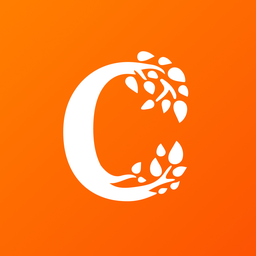
Full access? Get Clinical Tree
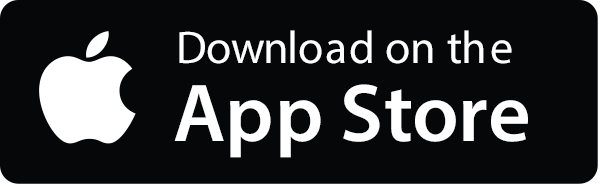
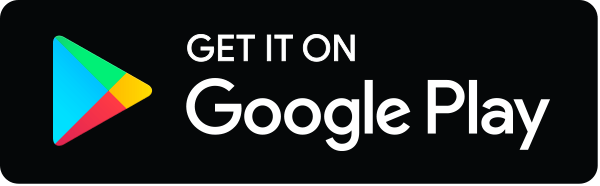
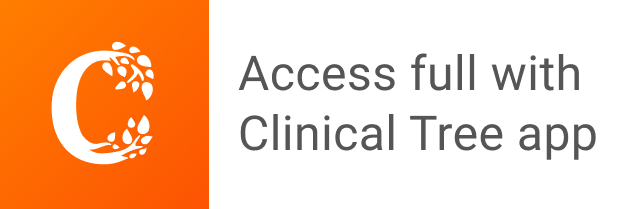