Abstract
Improvements in the intensive care and monitoring of patients with serious illnesses led to a greater appreciation of the significance of fluid movement into the lung as a complication of a variety of conditions. This, coupled with an improved understanding of the pathogenesis of pulmonary edema, has enhanced our ability to treat various illnesses in which pulmonary edema develops. The chapter first outlines the relevant anatomy, factors that control fluid movement within the lung, mechanisms responsible for the genesis of pulmonary edema, and how this edema fluid is cleared from the lung’s interstitium and airspaces. This background will enhance the reader’s understanding of the pathophysiologic consequences of edema formation, including the clinical and laboratory findings. Common disorders associated with pulmonary edema are then described along with the approach to therapy, which is based on understanding the underlying pathophysiologic mechanisms.
Keywords
pulmonary edema, review
Improvements in the intensive care and monitoring of patients with serious illnesses led to a greater appreciation of the significance of fluid movement into the lung as a complication of a variety of conditions. This, coupled with an improved understanding of the pathogenesis of pulmonary edema, has enhanced our ability to treat various illnesses in which pulmonary edema develops.
The chapter first outlines the relevant anatomy, factors that control fluid movement within the lung, mechanisms responsible for the genesis of pulmonary edema, and how this edema fluid is cleared from the lung’s interstitium and airspaces. This background will enhance the reader’s understanding of the pathophysiologic consequences of edema formation, including the clinical and laboratory findings. Common disorders associated with pulmonary edema are then described along with the approach to therapy, which is based on understanding the underlying pathophysiologic mechanisms.
Anatomic Considerations
Certain structural features of the lung are worth pointing out because they have a bearing on gas exchange during pulmonary edema. The capillaries are placed eccentrically within the alveolar septum ( Fig. 36.1A ). In some areas, the basement membranes of the capillary endothelium and the alveolar epithelium are fused with no additional space between them, even during edema formation. This situation is ideal for preserving gas exchange, at least until such time as the alveoli themselves are filled with liquid. In other areas, there is an interstitial space between the endothelial and epithelial basement membranes that contains secreted matrix. This matrix consists of structural proteins (e.g., collagens and elastin), attachment proteins for cells (e.g., laminin), and proteoglycans and glycosaminoglycans (e.g., hyaluronic acid, chondroitins, and heparan sulfate). In addition to supplying support to the capillary network, this widened portion of the alveolar-capillary membrane provides a channel for water and protein en route to the lymphatics and larger interstitial fluid spaces (see Fig. 36.1B ). As long as fluid can be confined to these channels, gas exchange can be preserved. Morphometric studies have shown that the majority of interstitial edema accumulates within the thick, and not the thin, portion of the alveolar-capillary membrane.

Pulmonary capillaries, similar to muscle capillaries, have a continuous endothelium with relatively tight intercellular junctions. The bronchial microvasculature, much like visceral capillaries, is discontinuous, with intercellular fenestrations or gaps. Whether these gaps account for the greater fluid movement across the bronchial capillaries, but not the pulmonary capillaries, remains to be determined. The role of bronchial circulation in the genesis of pulmonary edema may have been underestimated, but it is certain that bronchial circulation plays an important pathophysiologic role in inflammatory airway diseases such as asthma and inhalational lung injury.
At the ultrastructural level, the available evidence from tracer studies indicates that the alveolar epithelial membrane contains tighter intercellular junctions than does the capillary endothelial membrane. These epithelial tight junctions (zonulae occludentes) are estimated by both morphologic and physiologic studies to have an effective molecular radius of approximately 4 Å. This has two implications. First, they markedly restrict the movement of small ions, thereby making ions the most important solute in the genesis of osmotic pressure across the epithelial membrane. Second, fluid leaking from the vascular spaces is likely to be confined initially to the interstitial and lymphatic spaces; alveolar edema results only when the volume that can be handled by these spaces is overwhelmed. In contrast to the epithelium, the pores between endothelial cells have an effective molecular radius of approximately 40 Å. This 10-fold difference in interendothelial junction size allows the free movement of small ions and noncharged solutes, such as urea, but restricts the movement of larger macromolecules such as albumin and globulins. Thus, it is the protein concentration that is the most important solute in the genesis of transvascular osmotic pressure. These large macromolecules move across the microvasculature; however, the relative contributions of movement via pinocytotic vesicles (vesicular shuttle) versus across pores that can enlarge (“stretched pores”), especially when there is increased intravascular pressure or injury, is unclear.
Although the alveoli are often portrayed as spherical, a polyhedral model is closer to reality (see Chapter 6 ). From the perspective of fluid movement in the lung, the importance of this shape is that the walls of the alveolar septa are flat, except at the corners where the septa meet. Thus, it is only at the corners, where the alveolar air-liquid interface is curved, that the force exerted by surface tension can lower alveolar fluid and interstitial fluid pressures as predicted by the LaPlace relationship. The lower interstitial pressures at the corners favor movement of interstitial fluid that has traversed the alveolar capillary wall toward the corners of the alveolus. This is important from a lung fluid balance perspective. Alveolar type II epithelial cells are also predominately found in the corners of the alveoli.
Pulmonary blood vessels have been defined using both anatomic and physiologic criteria (see Chapter 6 ). Anatomically, the vessels have been classified traditionally by their morphologic characteristics as arteries, arterioles, capillaries, venules, or veins. Physiologically, these divisions are included under two broad classifications—alveolar and extraalveolar vessels—based on their behavior relative to the hydrostatic pressures of the interstitium surrounding the vessels, the interstitial fluid pressure. Alveolar vessels are in the alveolar walls and behave as if their outer walls were exposed to alveolar pressure. These vessels may collapse if airway pressures exceed vascular pressures, as is the case in zone I perfusion conditions. Extraalveolar vessels lie in the larger interstitial spaces and behave as if their outer walls were exposed to a pressure that is as negative as or more negative than pleural pressure and that tends to vary with pleural pressure. In addition, there are vessels lying in the intersections of alveolar septal walls (corner vessels) that are exposed to more negative pressures than the alveolar vessels. Unfortunately, there is no clear-cut correlation between the functional and the anatomic classifications. Fluid movement occurs at the level of the arterioles, capillaries, and venules; the bulk of fluid movement occurs at the level of the capillaries, with approximately three-fourths arising from the alveolar vessels.
Anatomically, the lung has two main compartments, each possessing markedly different potential volumes, into which edema fluid can move. The interstitial spaces of the alveolar capillary septae and peribronchovascular compartment can only accommodate small amounts of fluid. When this small interstitial safety reservoir, estimated to be a few hundred milliliters in an adult lung, is filled with edema fluid, subsequent accumulation must take place in the alveolar space, which at functional residual capacity (FRC) has a volume of approximately 30–40 mL/kg body weight or approximately 2000–3000 mL in the adult lung. This anatomic difference, in part, explains why interstitial edema may resolve quickly, whereas alveolar edema takes significantly longer periods of time.
Juxtacapillary (J) receptors are distributed throughout the lung’s interstitium and are stimulated by the presence of edema. They induce an increase in respiratory rate and their continued activation is responsible, in large part, for the continued tachypnea seen in pulmonary edema even when hypoxemia has been corrected through the use of supplemental oxygen and positive airway pressure.
Factors Responsible for Fluid Movement
The factors responsible for fluid accumulation include intravascular and interstitial hydrostatic and colloid osmotic pressures, permeability characteristics of the fluid-exchanging membrane, and lymphatic drainage.
The equilibrium of fluid across fluid-exchanging membranes is generally expressed as the Starling equation:
Q f = K f [ ( Pmv − Ppmv ) − σ ( π mv − π pmv ) ]
wherein, Q f = the net transvascular flow; K f = the hydraulic conductivity and filtration surface area of the fluid-exchanging vessels; Pmv = microvascular hydrostatic pressure; Ppmv = perimicrovascular (interstitial fluid) hydrostatic pressure; πmv = colloid osmotic pressure in the microvasculature; πpmv = colloid osmotic pressure in the perimicrovasculature, the interstitial fluid colloid osmotic pressure; and σ = the reflection coefficient, which is a measure of the resistance of the membrane to the movement of protein. Thus, σ influences the “effective” osmotic pressure of the protein. If the endothelium were completely impermeable to protein ( σ protein = 1), then the 5 g/dL of plasma would yield approximately 28 mm Hg osmotic pressure (each 1 mOsm/L of solute yields 19 mm Hg pressure). The osmotic pressure resulting from proteins is also termed the “oncotic pressure.”
As discussed subsequently, the absolute values for the variables within the Starling equation may change during health and disease. However, it should be emphasized that in the normal lung, Q f is positive and there is a continuous movement of fluid from the vascular to the interstitial spaces of the lung. Experimentally derived values are approximately: Pmv = 20 cm H 2 O, Ppmv = −2 cm H 2 O, πmv = −33 cm H 2 O, πpmv = 20 cm H 2 O, and 0.7 < σ < 0.95.
The term microvasculature is used to describe the vessels from which fluid leaks because fluid exchange is not limited to the capillaries alone. In the following discussion, each of the above factors and the pathophysiologic influences on them are described in detail.
Vascular Forces
The pressure in the pulmonary microvasculature (Pmv) is frequently, but not precisely, referred to as pulmonary capillary pressure. For technical reasons, this pressure is extremely difficult to measure in vivo. The pulmonary artery wedge pressure (Pw), also known as the pulmonary artery occlusion pressure, reflects the pressure in the first pulmonary veins where there is flow from nonobstructed vascular routes. Pmv is higher than left atrial (LA) pressure by approximately 40% of the difference between LA pressure and pulmonary arterial (PA) pressure. The relative amounts of arterial and venular resistance within the pulmonary vasculature is altered during hypoxia, the infusion of vasoactive agents (e.g., catecholamines) or during disease (e.g., endotoxinemia). An increase in either PA or LA pressure will tend to increase the hydrostatic pressure, favoring movement out of the fluid-exchanging vessels. For example, Pmv may be increased by the elevation in LA pressures in left-sided heart failure or by increases in PA pressure as seen in large left-to-right shunts. Although both PA and Pw pressures increase in a linear relation to exercise-induced increases in cardiac output, the ratio is significantly less than 1 : 1 in healthy young adult humans. Because the pulmonary vascular membrane is only slightly permeable to proteins and freely permeable to ions and small uncharged solutes, the plasma proteins normally are responsible for osmotic pressure (πmv). The πmv is significantly above the pulmonary microvascular hydrostatic pressure. The plasma colloid osmotic pressure may be markedly reduced in clinical conditions in which the plasma proteins are low (e.g., malnutrition, nephrosis, and massive burns) and thus may facilitate the formation of pulmonary edema.
Interstitial Forces
The interstitial hydrostatic pressure throughout the lung is normally negative relative to alveolar pressure, and there is a positive alveolar-hilar pressure gradient that facilitates the movement of interstitial fluid from alveolar to perihilar interstitial areas. The pressure surrounding the corner and extraalveolar vessels is less than pleural pressure and becomes considerably more negative at high lung volumes. In disease, these negative pressures may be amplified many fold because of “mechanical interdependence” of lung units. When the expansion of some units of the lung lags behind surrounding lung units because of disease, the force per unit area distending the lagging unit is increased. Amplification of transpulmonary (distending) pressures by mechanical interdependence is seen in conditions characterized by increased respiratory resistance, decreased lung compliance, and expansion of the lung from the airless state. Mechanical interdependence can act on diseased areas of the lung to produce distending pressures that are exceedingly high. When transmitted to the interstitial space around blood vessels, these pressures can enhance edema formation and can cause the rupture of vessels. These considerations become especially important, because various forms of constant distending pressures are used therapeutically. Although only 5–10 cm H 2 O may be applied, if the pressure does not distend some areas of the lung as quickly as others, the pressure surrounding lagging units may be considerably greater because of amplification.
Surfactant alters the liquid pressure within the airspace and, by extrapolation, the alveolar interstitial pressure. Thus increased air-liquid surface tension, whether as a result of inadequate or dysfunctional surfactant, will promote the movement of fluid from the vessels into the lungs.
Microvascular Filtration Coefficient and Vascular Permeability
There are significant technical difficulties in obtaining an accurate estimate of the K f within intact lungs and, dependent upon the species and experimental approach, estimates had varied by more than three orders of magnitude. However, when the experimental protocol ensures that there is full recruitment of the pulmonary vascular surface area, there is remarkable consistency of the normalized baseline K f values between species with widely varying body weights from mice to sheep. Because there is a similar relation between alveolar surface area and body mass of different species, this feature optimizes gas exchange.
Experiments have shown that the walls of the pulmonary circulation are not a perfect semipermeable membrane and that the normal pulmonary vasculature has 0 < σ < 1. The endothelial membrane has “pores” that are larger than some protein molecules. Fluid filtering through a pore will drag some protein with it. The larger the protein relative to the size of the pore, the less protein will be dragged. When the protein is the same size as or larger than the pore, the reflection coefficient σ is 1. As the size of the protein becomes progressively smaller, σ approaches zero.
Early experimental evidence suggested that the microvascular permeability to protein is greater in the young than in the adult animal; however, subsequent work showed that there was no difference in lung microvascular permeability to protein between late-term and postnatal animals and that the higher rate of fluid movement out of the newborn lung’s microvasculature bed likely results from a greater portion of the younger smaller lung being in West’s zone III perfusion status.
Lymphatic Clearance
Whether there is fluid accumulation in the lung depends on the balance between fluid filtration into the lung and lymphatic clearance. Early in the onset of interstitial edema, lymphatic drainage of fluid is an important protective mechanism to prevent alveolar flooding. Although early work had indicated that increased motion or ventilation of the lung increased the lymphatic fluid drainage, suggesting a passive milking action, it is now known that there are active contractions of the lymphatic smooth muscle that can be further augmented by vasoactive agents. Indeed, rhythmic inflation and deflation is not required for normal lymphatic function in lungs that have normal or increased vascular permeability. Lung lymph flow can increase up to tenfold acutely, and when there is chronic edema, the maximal ability of the lymphatics to clear fluid may increase many fold, presumably as the result of proliferation of the lymphatic vasculature. Because the lymphatics ultimately drain into the great veins, elevation of systemic venous pressure might be expected to increase fluid accumulation, not only by raising pressure in the fluid-exchanging vessels, but also by opposing lymphatic drainage.
Surface Tension
Surface tension at the air-liquid interface on the inner surface of the alveolus tends to pull fluid away from the alveolar epithelium with a force of at least 2 mm Hg. This surface tension at the alveolar air-liquid interface would be expected to expand the perivascular space and to lower perimicrovascular pressure. As pulmonary edema fluid enters the airspace, it first collects in the corners, but as fluid continues to accumulate, the filling of an alveolus with fluid is self-accelerating once there is a critical amount of fluid present.
Safety Factors That Oppose Edema Formation
A variety of clinical observations have indicated that transvascular hydrostatic pressures must be raised by 15–20 mm Hg before edema develops. Several factors provide this protection against edema formation. The interstitial fluid pressure, as previously described, is below alveolar pressure and will rise when there is even minimal amounts of fluid accumulation within the lung and thus oppose fluid movement. At the same time, the filtered fluid will dilute the interstitial plasma protein, thus lowering the interstitial colloid osmotic pressure and diminishing the movement of fluid out of the microvasculature. Lymphatic drainage of fluid and protein also contributes to this “margin of safety.” The interstitial space itself, especially around the bronchi and blood vessels (bronchovascular cuffs), can sequester fluid (several hundred milliliters in the adult) and thus can provide an additional safety factor before fluid floods the alveoli.
Mechanisms That Cause Pulmonary Edema
There has been much effort to classify the different causes of pulmonary edema into cardiogenic and noncardiogenic pulmonary edema. Although this is useful to some degree, it should be remembered that in many lung diseases characterized by pulmonary edema, there are both increased transvascular pressure gradients and increased permeability to solutes. For example, 30% of patients diagnosed with acute lung injury (ALI) have a pulmonary artery wedge (occlusion) pressure greater than 18 mm Hg. The various etiologies of pulmonary edema are introduced by using the Starling equation as the basis for the discussion.
Increased Hydrostatic Pressure in the Pulmonary Microvasculature
Increased hydrostatic pressure in the Pmv is the most common and perhaps most easily understood cause of pulmonary edema in the pediatric and adult population. A variety of clinical conditions are associated with increased hydrostatic pressures in the Pmv, either as the result of elevation of vascular pressures distal to the lung’s parenchyma, increased blood flow, increased blood volume, or PA or venular hypertension. In each case, there would be an increase in the amount of water and solute leaving the microvasculature and entering the interstitium. Although hypoxia increases PA pressures, it does not by itself increase vascular permeability to solutes.
Decreased Plasma Colloid Osmotic Pressure
If a patient has no other disorders, hypoproteinemia will not, by itself, cause pulmonary edema. Large pleural effusions may develop in diseases such as the nephrotic syndrome, but there is no evidence of lung edema as assessed by gas exchange and chest radiography. However, in patients where vascular pressure or alveolar capillary membrane permeability increases, pulmonary edema is more likely to develop and be more severe when the plasma protein concentration is low. This is seen in various conditions including severe malnutrition, massive burns, protein-losing enteropathies, and nephrosis. Hypoproteinemia also can be seen in patients with a variety of other conditions when withdrawal of multiple blood samples for diagnostic purposes is coupled with the administration of large amounts of noncolloid-containing fluids.
Decreased Interstitial Hydrostatic Pressure
As a result of mechanical interdependence of adjacent lung units, when inflation of some units lags behind that of others, large negative interstitial pressures can be generated around and within the lagging units. These negative pressures can be transmitted to the fluid-exchanging vessels (when there is airway closure), enhancing edema formation, especially in obstructive lung diseases such as asthma, bronchiolitis, and bronchopulmonary dysplasia (BPD), as well as in nonobstructive “stiff lung” disorders such as the respiratory distress syndrome (RDS).
Increased Pulmonary Vascular Surface Area
The normal adult lung usually has approximately one-third of its microvascular bed perfused under resting conditions. Infants and young children have a greater percentage of their vascular bed distended with blood. Regardless of the absolute percentage, the lungs of all age groups can undergo significant recruitment and distention of the pulmonary vasculature. Although it had been suggested that various regions of the normal lung might have different permeability to solutes, most studies suggest that lung fluid movement simply increases in direct proportion to the increase in perfused pulmonary vascular surface area. Under normal conditions, the lung lymphatics can easily accommodate the threefold to fourfold increase in lung water and solute movement that is associated with full recruitment of the vasculature. However, when pulmonary vascular permeability is increased, similar amounts of recruitment can lead to marked increases in fluid movement as vessels with high permeability are recruited.
Increased Vascular Permeability in Fluid-Exchanging Vessels
Increased alveolar-capillary membrane permeability to solutes can occur by different routes. Classic modeling of the Pmv uses the concept of various sized “pores” through which various-sized solutes move under normal physiologic conditions. Thus, permeability could increase via an increase in the total number of pores, the diameter of the pores, or a combination of the two phenomena. For example, vasoactive agents could increase the size of the interendothelial junctions. Alternatively, or in addition, direct and extensive damage to the alveolar epithelium or endothelium occurring during overventilation lung injury or stress-induced endothelial injury will open up large nonphysiologic pathways for fluid and solute movement.
A variety of clinical conditions are believed to alter the permeability of the alveolar capillary membrane, presumably by damage to epithelial and endothelial cells. The cellular mechanisms for this injury can be divided into two major categories: direct and inflammatory-mediated lung injury.
Direct lung injury can occur when a toxic substance directly causes cell injury without a preceding inflammatory response. Direct lung injury is seen during the inhalation of a variety of noxious gases, including the oxides of sulfur and nitrogen, hydrocyanic acid, and aldehydes. Similarly, the inhalation of gastric acid can directly damage lung epithelium. These produce denaturation of proteins, cellular damage, and pulmonary edema.
Inflammation-mediated lung injury, as occurs in ALI and acute respiratory distress syndrome (ARDS), most frequently arises from leukocytes and their products. The unregulated release of leukocyte-derived toxic products occurs in response to direct injury or in response to various infective and inflammatory stimuli. These toxic products include reactive oxygen species (e.g., superoxide, hydrogen peroxide, hypochlorous acid, hydroxyl radical, peroxynitrite), proteolytic enzymes (e.g., elastase, collagenase, lysozyme), products of arachidonic acid (e.g., platelet-activating factor), and cationic proteins. Leukocytes have been implicated in animal models of ALI induced by the administration of endotoxin, hyperoxia, microembolization, and mechanical ventilation. Nonleukocyte-derived vasoactive mediators also can increase vascular permeability. Examples include histamine, prostaglandins, cytokines, proteases, and reactive oxygen intermediates.
Increased permeability of the microvasculature also occurs in a variety of disorders characterized by aberrant regulation of the immune system. These include hypersensitivity pneumonitis and pulmonary vasculitis, which can occur in various disorders such as acute pulmonary systemic lupus erythematosus.
When there is increased pulmonary vascular permeability, an increase in microvascular hydrostatic pressure or pulmonary blood flow produces a much greater outward flow of fluid. As such, the combination of increased permeability and high LA pressures represents an especially difficult clinical challenge.
Clearance of Pulmonary Edema Fluid
Once the basic condition producing the edema is reversed, how quickly pulmonary edema resolves depends on whether the fluid is confined to the interstitium, from which it can be cleared in hours, or is also located in the alveolar space, from which it may take many hours or days to clear.
Interstitial fluid has two pathways for clearance. The lymphatics play the most important major role; however, a second site is the venular end of the microvascular bed where Pmv has decreased and the balance of Starling forces can favor reabsorption. Alveolar edema fluid, after being actively transported across the epithelium, is returned to the circulation either by direct entry into the Pmv across the thin side of alveolar capillary membrane or by the lymphatics after it has been translocated back to the interstitial space. Both of these potential pathways, however, require water and solutes to have first traversed the distal lung epithelium.
In both high-pressure and high-permeability pulmonary edema, there is a substantial amount of protein within the alveolar fluid that opposes protein osmotic reabsorption, and it has been shown that passive forces cannot explain the clearance of alveolar fluid from the intact lung. Long-term studies have demonstrated that as edema fluid is reabsorbed, the protein concentration in the alveolar space actually increases to levels above those in the plasma. This suggests that an active transport of salt and water was involved in the clearance of airspace fluids. Numerous studies, as reviewed recently, have demonstrated that nonprimate mammalian and human distal lung epithelium actively transports Na + with Cl − and water following ( Fig. 36.2 ). Although most research, for reasons of feasibility only, have focused on the alveolar type II epithelium’s ability to actively transport salt, it is known that both the alveolar type I epithelium and Clara cells play important roles in this active epithelial Na + transport. Studies in adult patients have indicated that active alveolar fluid clearance rates in the human are in the range of 25% per hour.

Protein clearance from the alveolar spaces is significantly slower than salt and water, and in animals, it is in the range of 1% per hour. The relative amount of protein cleared by metabolic degradation and macrophage ingestion versus active transport by the pulmonary epithelium is unknown, but active transport is involved in the clearance of at least some proteins from the airspaces of the lungs. Protein clearance from the interstitial space is believed to occur primarily by lymphatic clearance, but direct penetration into the circulation either before or after metabolic degradation might also occur.
The presence of protein in the alveolar and interstitial spaces may attract inflammatory cells. In ALI syndromes, there is marked leakage of plasma protein into the alveolar space, and resultant activation of the coagulation cascade results in fibrin formation. The coagulum opposes the efficient reabsorption of fluid, and fibrin and fibrin degradation products are potent stimuli for fibrosis within the lung. Circulating monocytes and alveolar macrophages likely play a major role in the clearance of inflammatory edema protein from the lung’s airspaces.
In summary, a wide variety of mechanisms and sites exist for protein and electrolyte clearance and fluid removal, including pulmonary and bronchial circulations, lymphatics, active transport of ions, macromolecular metabolism and degradation, and mononuclear cell activity.
Pathophysiologic Consequences of Edema
The pathophysiologic consequences and clinical findings in pulmonary edema are best understood by reviewing the sequence of events that lead from interstitial to airspace edema. Edema accumulates within the lung in a step-wise fashion ( Fig. 36.3 ). Distal lung units in different regions of the lung will be at different stages of fluid accumulation because of their regional differences in pressure, alveolar-capillary membrane integrity, and gravitationally dependent factors.
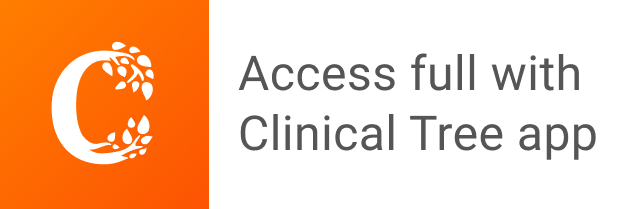