Principles of Oxygenator Function: Gas Exchange, Heat Transfer, and Operation
Michael H. Hines
INTRODUCTION
Since the early 1950s when the development of a heart-lung machine first began, there has been a tremendous evolution of devices and machinery for cardiac support (1,2). However, despite the diversity in designs through the years, they all contain three essential components: a mechanism to circulate the blood, a method of gas exchange for oxygen and carbon dioxide, and some mechanism for temperature control. Chapter 2 has covered the first important component, and we will now focus on the two subsequent elements: gas exchange and heat transfer. And while it is referred to as the “oxygenator,” we must recognize that it is responsible for the movement of both oxygen in, as well as carbon dioxide out. The discussion will start with a basic review of the principles of physics, and then we will apply those principles to the devices used specifically in extracorporeal support, including cardiopulmonary bypass (CPB) and extracorporeal membrane oxygenation (ECMO).
You may notice as you go through this chapter that there is a scarcity of trade and manufacturer names. The author has intentionally avoided using these. The intent was primarily to focus on the physiology, physics, and chemistry of the oxygenator and heat exchanger, but also to emphasize the fact that there are a large number of manufacturers producing many products that have all been shown to function extremely well. While we have witnessed dramatic improvements with the evolution from bubble to membrane to hollow fiber oxygenators, there is little, if any, data demonstrating definitive superiority of one product over another within one class, for example, polypropylene hollow fiber oxygenators. In fact, many comparisons have demonstrated only subtle differences that have minimal impact on clinical practice (3). This includes the multitude of different coatings which we will discuss using generic chemical names. We have on occasion provided references that have specifically compared different products such as biocompatibility coatings, but will not include this information in the text. In doing this, we can more easily remove any commercial bias, and hope to offer the reader a better understanding of the principles and functionality of the devices so that they can then evaluate the available commercial options themselves and select products that best suit their needs based on applications, availability, margins of safety, cost, and track records.
PHYSICS OF GAS EXCHANGE
The movement of gas molecules of oxygen (O2) and carbon dioxide (CO2) between air and blood across a biologic or synthetic barrier is controlled by several specific laws of physics. We will first review the principles of those laws and then discuss their direct application to the natural process in the human lung, as well as our attempt to imitate the natural process with various devices and techniques.
Dalton’s Law (John Dalton, 1801):

The total pressure of a mixture of gases is equal to the sum of the partial pressures of all the individual gases in that volume. At sea level this must equal 760 mm Hg.
Fick’s First Law of Diffusion (Adolf Fick, 1855):
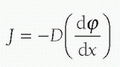
In this mathematical formula, J represents the diffusion flux or amount of substance (e.g., O2) moved per unit area, per unit time. D, the diffusion coefficient, is a constant for the particular barrier, based on its composition, and so forth. It is also referred to as the “diffusivity,” and the preceding negative sign simply makes the flux J positive when the movement is down the concentration gradient. The diffusion coefficient for specific molecules of gas across biologic membranes such as the alveoli may change relatively rapidly due to inflammation, edema, or injury that may change the characteristics of the membrane. However, it should be constant for synthetic barriers such as membrane oxygenators, affected only by significant changes in temperature and pressure (or at least until it is placed in the biologic setting when it can be affected by clotting, etc.). The substance concentration is represented by φ and the length by x. For purposes of the discussion of gas transfer across membranes, Fick’s first law of diffusion tells us that the movement of O2 and CO2 across a barrier will be in the direction of higher to lower concentration (partial pressure) with a magnitude that is proportional to the gradient, and proportional to the area involved, and inversely
proportional to the diffusion coefficient or “diffusivity.” Simply put, gas diffusion occurs faster when the gradient across the membrane is higher, and a “thinner” barrier allows more diffusion of gas than a “thicker” one, while a barrier of equal “thickness” but much larger surface area will allow more gas to diffuse during the same time interval.
proportional to the diffusion coefficient or “diffusivity.” Simply put, gas diffusion occurs faster when the gradient across the membrane is higher, and a “thinner” barrier allows more diffusion of gas than a “thicker” one, while a barrier of equal “thickness” but much larger surface area will allow more gas to diffuse during the same time interval.
Graham’s Law (Thomas Graham, 1848):
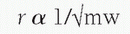
The diffusion rate of a gas is inversely proportional to the square root of its molecular weight.
Henry’s Law (William Henry, 1803):
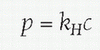
The amount of gas that can dissolve in a volume of liquid is directly proportional to the partial pressure of the gas in that liquid. Mathematically, where p is the partial pressure of a particular gas, c is the concentration of the dissolved gas, and kH is a constant for a particular gas in a particular solution; for example, kH for O2 dissolved in water at 298 K is 769.2 L atm/mol. It is this principle that allows gas bubbles to come out of solution in the blood during depressurization, as when scuba divers get “the bends” from too rapid an ascent from diving. Henry’s law allows larger quantities of gases inhaled at depths under much higher partial pressure due to the surrounding water to become dissolved into solution in the blood, particularly with longer time periods of exposure. If the diver ascends rapidly before the lungs are able to gradually expel the absorbed gas, then the excess gas forms air bubbles in the blood, which are responsible for the symptoms and organ damage of decompression illness, also known as “the bends” or Caisson disease.
Principle of Countercurrent Exchange
Given two parallel tubes filled with fluid or gas separated by a membrane with some degree of permeability to components of that fluid or gas, the exchange of molecules or particles across the barrier is more efficient if the movements of the liquids are opposite in direction. In a theoretical example with a readily diffusible gas G that we want to move from one system to another through diffusion, we will assume that the concentration of G in what we will refer to as the “donor” system is 100%, and that there is zero amount of G in the “recipient” side of the system (Fig. 3.1). If the two systems are moving in parallel (concurrent), then at the entrance the concentrations are 100% and 0%. As the two systems move along, there is continued diffusion of G across the barrier with reduction of the concentration of G on the donor side, and increase in the concentration on the recipient side. Gradually the concentrations change to 90%:10%, 80%:20%, and so on until equilibrium is reached at 50%:50%. Continued movement along the additional length of the tube does not provide any additional exchange of G into the recipient system, and the end result at the exit site remains 50%. If we now reverse the direction of one of the systems so that the donor and recipient systems are flowing in opposite directions (countercurrent), the movement of G can occur throughout the entire length of the system, because the gradient driving the diffusion can be maintained. If there is sufficient length to the systems, the concentration of G can potentially reach 100% at its exit site, immediately adjacent to the inlet side of the donor system
where the concentration is also 100%. Thus the equilibrium is reached at 100% rather than 50%. The gas exchange across the gills of fish is an example of the natural biologic application of this efficient principle of countercurrent exchange. Countercurrent exchange applies not only to the transfer of gases as in the example, but equally to movement of substances between liquids, a gas and a liquid, as well as to transfer of heat between two fluids (liquids or gases).
where the concentration is also 100%. Thus the equilibrium is reached at 100% rather than 50%. The gas exchange across the gills of fish is an example of the natural biologic application of this efficient principle of countercurrent exchange. Countercurrent exchange applies not only to the transfer of gases as in the example, but equally to movement of substances between liquids, a gas and a liquid, as well as to transfer of heat between two fluids (liquids or gases).
OXYGENATORS FOR EXTRACORPOREAL SUPPORT
Basic Oxygenator Design
The natural process of gas exchange in the lung involves directing the blood into small capillaries adjacent to the thin-walled alveolus containing inhaled air so that oxygen may diffuse in and carbon dioxide may diffuse out, based on the principles of diffusion in Fick’s law outlined above. The capillary size is such that the cells move through essentially one at a time to provide maximal exposure and time for gas exchange. The massive respiratory surface area of the normal human lung provides for extremely efficient and large-volume exchange of O2 and CO2. Appropriate exchange does require the efficient movement of air in and out of the alveoli through the same passages, which creates the potential for dead space ventilation issues.
In designing an artificial lung equivalent to be used for short periods during CPB, many factors must be considered which frequently play against each other. For example, having a very large surface area will maximize the potential for gas exchange, but then also increase the size of the device, and thus the priming volume required to fill it, and the associated hemodilution and potential transfusion requirement. Mimicking the natural lung with tiny “capillary-like” blood passages would also maximize the efficiency of exchange, yet would create extremely high resistances within the membrane, requiring a very large surface area, and leading to more shear stresses, hemolysis, and so forth. Thus, the development of the ideal device for gas exchange has been built upon a series of compromises between the advantages and disadvantages of available surface area, resistance to flow, size, priming volume, diffusion capability, plasma leakage, and biocompatibility.
Measurements of Oxygenator Performance and Capabilities
In an attempt to create industry standards for comparison of capabilities between oxygenators, the Association for the Advancement of Medical Instrumentation previously developed a set of standards for oxygenators. However, the reliability of those standards was questioned because they failed to consider the impact of variability in inlet conditions which affects the variability of gas transfer. It was subsequently shown that improvements in gas transfer in some devices could be demonstrated by changing the conditions under which the oxygenators were evaluated (4,5,6). Despite the lack of acceptance of those standards, there are a series of measurements that help to define the physical and functional capacity of oxygenators. As previously referred to, the priming volume is the volume of fluid (crystalloid or blood) required to fill the blood phase of the device, including any integrated heat exchanger. This may also include some minimal level in the reservoir depending on the device and the manufacturer. Manufacturers also provide minimum and maximum operating volumes for their specific devices. The ability of an oxygenator to oxygenate blood is expressed in the oxygen reference blood flow, which is defined as the flow rate of whole blood at normothermia, with a normal hemoglobin (12 g/dL), with a base excess of zero, that will increase the oxygen content of venous blood with an oxygen saturation of 65%, by 45 mL/L of flow. Similar measurements of a device’s ability to remove CO2 are expressed in the carbon dioxide reference blood flow. Also provided is the index of hemolysis for the device, expressed as the amount of free hemoglobin/100 cc of blood pumped through the oxygenator during an in vitro test. Finally, the rated flow or reference flow is the maximal recommended flow to achieve adequate gas exchange, and is equal to the lowest flow among the oxygen reference blood flow, carbon dioxide reference blood flow, manufacturer’s recommended maximal flow, or 8 L/min (7).
Direct Contact Oxygenators (Bubble, Screen, Rotating Disc, Drum)
Without repeating the history of the development of the early-generation oxygenators covered in the previous chapters of this book, we will discuss only the physiologic concepts of gas exchange where there is direct contact of blood with the gas phase, rather than the specifics of the designs of the various early machines. In all of the devices, the blood from the patient’s body would enter the machine and at some point directly mix with a gas mixture of primarily oxygen in the form of very small bubbles generated through several mechanisms, frequently using a device called a sparger that generated the gas bubbles (8). Each bubble provided the surface area within the blood for gas exchange. The size of the bubbles became very important, since the available surface area for gas exchange is inversely proportional to the bubble diameter: smaller bubbles provided more surface area and more efficient transfer of oxygen. However, if the bubbles were too small, the rapid rise in CO2 tension limited the amount of CO2 removal that was possible. Gradually, manufacturers determined the proper-sized bubbles that would provide an ideal respiratory quotient for the system of about 0.8 (CO2 elimination: O2 uptake). Once the bubbles are generated and mix with the venous blood, the mixture has sufficient time for the transfer of oxygen directly onto the hemoglobin molecules in the blood (with a very small proportion going into solution). Simultaneously, the CO2 would leave the solution and enter the bubbles, exiting near the top of the device or in a separator. As the blood progresses, any residual bubbles would gradually coalesce and be filtered from the blood prior to it being returned
to the patient. One of the biggest hurdles with this design was the formation of foam from the aeration of the blood, and various de-foaming agents and designs were used to try to minimize this problem. A major advantage was the very efficient gas exchange that the direct contact provided. However, the same direct exposure was also severely injurious to the cellular blood components if used for prolonged periods. Another advantage was the very low pressure drop or resistance in the bubble oxygenator design, since there was no need to direct the blood through small conduits adjacent to the gas phase for O2 and CO2 exchange as in a membrane. The driving force of the venous blood was simply the hydrostatic column, and its upward motion during the gas exchange was facilitated by the rising bubbles. The large size and capacitance of the devices meant they could also serve as the venous reservoirs for the system.
to the patient. One of the biggest hurdles with this design was the formation of foam from the aeration of the blood, and various de-foaming agents and designs were used to try to minimize this problem. A major advantage was the very efficient gas exchange that the direct contact provided. However, the same direct exposure was also severely injurious to the cellular blood components if used for prolonged periods. Another advantage was the very low pressure drop or resistance in the bubble oxygenator design, since there was no need to direct the blood through small conduits adjacent to the gas phase for O2 and CO2 exchange as in a membrane. The driving force of the venous blood was simply the hydrostatic column, and its upward motion during the gas exchange was facilitated by the rising bubbles. The large size and capacitance of the devices meant they could also serve as the venous reservoirs for the system.
Despite the physiology simplicity of the bubble oxygenators and the other direct contact devices, the cost and complexity of the setup, along with the limitations caused by direct damage to the blood, made it less practical for longer or more complex cardiac operations, and led to the development of more sophisticated oxygenators.
Membrane Oxygenators
Many different materials were trialed in the early designs of gas exchange devices, including ceramics, plastics, rubber, and a number of synthetic products like cellulose, polyethylene, and Teflon. During this time, much understanding was gained in the mechanisms of gas exchange across a membrane oxygenator (9). However, the first widely applicable clinical oxygenator which completely eliminated the direct contact of the blood and gas phases, making it a true membrane oxygenator, was designed by Kolobow and colleagues (10,11). The advantages the new membrane offered were the separation of the blood and gas, reducing the damage and thrombosis seen with bubble oxygenators (12), as well as the reduction of gaseous emboli (13). In addition, with the new oxygenators, the blood was no longer pushed upward by the moving gas bubbles, but was pumped through the membrane independent of the gas flow, thus allowing separate regulation of the rate and composition of the gas phase to manage O2 and CO2 exchange. The membrane was constructed as a long rolled coil from a sheet of a silicone polymer which completely divided the gas and blood phases. Gas exchange was not as efficient as through other materials, in that the oxygen had to essentially diffuse into the silicone polymer phase and then diffuse out into the blood, with the same process in reverse for carbon dioxide. Thus, much larger surface areas were required and concurrently larger priming volumes. The membrane also had relatively high resistance or pressure drop from the inlet to outlet of the blood phase. The membrane had acceptable biocompatibility, requiring significant anticoagulation and was recognized to stimulate the fibrinolytic system, and was also found to absorb large quantities of certain drugs, making therapeutic levels often difficult to achieve. However, unlike other synthetic plastics in use for clinical oxygenators, primarily polypropylene, the silicone membrane remained essentially impermeable to plasma proteins and could often be used for weeks without failure, making it ideal for long-term support such as ECMO. In the operating room where the oxygenator was required to be functional for only few hours, and where plasma leakage and oxygenator failure were less of an issue, the large size and priming volumes made it much less practical than other available options.
Microporous Oxygenators
Unlike the large, less efficient silicone membrane oxygenators, the so-called microporous hollow fiber oxygenators were designed specifically for the needs of the operating room where short-term use with small devices requiring low priming volumes and low resistances were very advantageous. The vast majority of oxygenators were made of polypropylene hollow fibers, although a few utilized sheets of polypropylene, where micropores less than 1 ìm are created through a process of heating and stretching the material. The gas moves through the small fibers which are surrounded by blood moving in countercurrent fashion. Once exposed to blood, the pores in the fibers become coated with plasma proteins through which gas molecules may pass, but through which the plasma proteins and water do not due to the surface tension of the blood. Over time, the pores eventually allow plasma components across the pores into the gas phase, known as plasma leakage. This usually takes a number of hours or even days, much longer than needed for CPB support; so it is usually not clinically relevant, except when these membranes are used for longer-term support such as ECMO. In those cases, very close monitoring of oxygenator function and frequent oxygenator changes are required.
While the goal of the oxygenator is to mimic the function of the native lung by providing sufficient oxygen supply and carbon dioxide removal, it is not feasible to reproduce the structure of the lung. Gas flow moves across the oxygenator instead of in and out of airways, which provides some advantage for oxygenator efficiency, perhaps compensating for the fact that the oxygenator cannot begin to reproduce the lung model of gas exchange. Oxygen-rich air enters the alveoli with inspiration and spends a short time within the massive respiratory surface area that is the lung. Adjacent to the millions of alveoli are a similar number of tiny capillaries so small that the red blood cells (RBCs) line up one by one to pass through as they perform the required exchange of gas molecules. The hollow fiber oxygenators cannot begin to compete with such an efficient system. However, with additional understanding of fluid dynamics and gas exchange, engineers have been able to design remarkably efficient devices by compensating for weaknesses with additional strengths. This involves extremely complex physics of fluid dynamics, which has been explained in detail elsewhere (14), and which we will try to simplify here for the application of oxygenators. We noted earlier that the smallest feasible constructible pathway for blood is still
over 100 times the size of capillaries, and if made any smaller the resistance becomes prohibitive for the purposes of extracorporeal support. This is compensated for by increasing the length of the blood path over 1,000-fold. So instead of each cell briefly passing along an individual alveolus, we now have larger amounts of blood passing through wider, but very long distances to create more effective surface area for gas exchange. Because the blood is a viscous fluid, the velocity of the flow is not consistent everywhere within the oxygenator. And while the gas actually flows within the microfibers, and the blood around them, let us consider the movement of blood as if it were in a tube, to get a better understanding of the fluid dynamics and its impact on gas exchange. Within the “tube,” the velocity of the blood varies, with the fastest flow in the center and the surrounding blood moving at gradually decreasing velocities as it gets further from the center and closer to the outer edge, which would be the interface with the gas phase. At this point, the velocity is theoretically zero and the central flow is maximal. At the interface where there is zero velocity, a boundary layer is formed where diffusion of oxygen takes place across the membrane. Since the solubility of oxygen into the liquid portion of blood is very low, the majority of diffusion occurs at or near the interface onto the hemoglobin within the blood near the boundary layer. Diffusion further into stream is more difficult because of distance. If the length of the blood path is short and there is perfectly laminar flow, little to no oxygen would get to the hemoglobin in the central stream of blood. However, the flow is not laminar and turbulence acts to disrupt the layers of the velocity gradient and increases eddy currents and mixing. In this circumstance, this is highly desirable so as to increase the ability of oxygen to diffuse onto more available hemoglobin molecules. This problem could be also solved by increasing the blood path length and dwell time, but this would dramatically increase the surface area, the size of the oxygenator, and thus the prime volume. Another solution would be to decrease the width of the blood path, reducing the distance between the interface and the central stream, but as previously stated, this would significantly increase the resistance to flow to an impractical level. By putting the gas through the fibers instead of the blood, the resistance is much lower and allows adequate contact for gas exchange. The gas-containing microfibers are placed in nonlinear fashion with a number of gentle twists and turns creating turbulence and increased mixing, but not so much as to increase shear stress and cause damage to the RBCs. Additional fans or small blades are placed within the blood phase to achieve the same effect, which is known as “secondary flow” (15).
over 100 times the size of capillaries, and if made any smaller the resistance becomes prohibitive for the purposes of extracorporeal support. This is compensated for by increasing the length of the blood path over 1,000-fold. So instead of each cell briefly passing along an individual alveolus, we now have larger amounts of blood passing through wider, but very long distances to create more effective surface area for gas exchange. Because the blood is a viscous fluid, the velocity of the flow is not consistent everywhere within the oxygenator. And while the gas actually flows within the microfibers, and the blood around them, let us consider the movement of blood as if it were in a tube, to get a better understanding of the fluid dynamics and its impact on gas exchange. Within the “tube,” the velocity of the blood varies, with the fastest flow in the center and the surrounding blood moving at gradually decreasing velocities as it gets further from the center and closer to the outer edge, which would be the interface with the gas phase. At this point, the velocity is theoretically zero and the central flow is maximal. At the interface where there is zero velocity, a boundary layer is formed where diffusion of oxygen takes place across the membrane. Since the solubility of oxygen into the liquid portion of blood is very low, the majority of diffusion occurs at or near the interface onto the hemoglobin within the blood near the boundary layer. Diffusion further into stream is more difficult because of distance. If the length of the blood path is short and there is perfectly laminar flow, little to no oxygen would get to the hemoglobin in the central stream of blood. However, the flow is not laminar and turbulence acts to disrupt the layers of the velocity gradient and increases eddy currents and mixing. In this circumstance, this is highly desirable so as to increase the ability of oxygen to diffuse onto more available hemoglobin molecules. This problem could be also solved by increasing the blood path length and dwell time, but this would dramatically increase the surface area, the size of the oxygenator, and thus the prime volume. Another solution would be to decrease the width of the blood path, reducing the distance between the interface and the central stream, but as previously stated, this would significantly increase the resistance to flow to an impractical level. By putting the gas through the fibers instead of the blood, the resistance is much lower and allows adequate contact for gas exchange. The gas-containing microfibers are placed in nonlinear fashion with a number of gentle twists and turns creating turbulence and increased mixing, but not so much as to increase shear stress and cause damage to the RBCs. Additional fans or small blades are placed within the blood phase to achieve the same effect, which is known as “secondary flow” (15).
Unlike oxygen, carbon dioxide is much more soluble in blood where it is quickly converted to bicarbonate. Additional molecules of CO2 are transported on amine groups of plasma proteins, including hemoglobin, making the excretion of CO2 much less problematic than the delivery of oxygen (Figs. 3.2 and 3.3).
“Plasma-Tight” Hollow Fiber Oxygenators
In 2008, the first polymethylpentene (PMP) hollow fiber oxygenator was approved by the Food and Drug Administration (FDA) for use in the United States for extracorporeal support. While PMP oxygenators had been used in Europe for many years, their introduction in the United States provided an important new tool for long-term extracorporeal support. Like the polypropylene predecessor, the PMP oxygenators had very efficient gas exchange, low surface areas and priming volumes, excellent biocompatibility, and very low resistance to blood flow. However, the hollow fibers of PMP were truly nonporous, instead of being covered with a very thin membrane, which allowed efficient gas exchange without the eventual plasma leakage, although rare cases have been reported (16). While this made little difference in the operating theater, it revolutionized ECMO support in this country and around the world by providing a low-volume, low-resistance, biocompatible, and efficient oxygenator that could be used for days or weeks at a stretch (Fig. 3.4). The only disadvantage this author has noted with these oxygenators in comparison to the
previously employed silicone membranes is the rapidity with which they fail when they do get thrombosis. While the stimulus for coagulation does seem to be less with the PMP and its various coatings, because the surface area and volume are so small, when thrombosis is initiated, it can progress extremely rapidly causing relatively sudden oxygenator failure. This becomes extremely important in light of the trends in ECMO support and the desire for a simpler and more “automated” and compact system, which has led to less monitoring of pre- and postmembrane pressures and less close observation by experienced staff. With that comes less warning of potential problems, and perhaps less safety margin in preventing a sudden and potentially catastrophic failure of the system.
previously employed silicone membranes is the rapidity with which they fail when they do get thrombosis. While the stimulus for coagulation does seem to be less with the PMP and its various coatings, because the surface area and volume are so small, when thrombosis is initiated, it can progress extremely rapidly causing relatively sudden oxygenator failure. This becomes extremely important in light of the trends in ECMO support and the desire for a simpler and more “automated” and compact system, which has led to less monitoring of pre- and postmembrane pressures and less close observation by experienced staff. With that comes less warning of potential problems, and perhaps less safety margin in preventing a sudden and potentially catastrophic failure of the system.
Reservoirs
Systems for CPB also include some form of reservoir—either a soft-collapsible device or a solid container that serves a number of functions, or some combination (see Figs. 3.2 and 3.3). The primary function is the regulation of volume and provides the pump a continued source of blood for delivery to the patient, even if there is temporary interruption of venous return from the patient, either intentional or inadvertent. The reservoir is made large enough to completely exsanguinate the patient’s blood volume in cases of deep hypothermic circulatory arrest. After entering the reservoir, the blood spends a short amount of time there, allowing any bubbles that may have entered to rise to the top, as the blood is then drained from the bottom of the reservoir to be then pumped through the oxygenator and heat exchanger. This transient stasis is very useful in de-airing the blood, particularly with the use of suction devices that return shed blood directly back to the pump, but is also the reason that CPB requires a higher level of anticoagulation (usually activated clotting times over 400 seconds) when compared to ECMO circuits which have no reservoir, minimal areas of stasis, and can be run with much lower levels of anticoagulation. But because the ECMO circuits do not have any reservoir (other than a small servo-regulator or compliance chamber), their continued function is completely dependent on a steady and uninterrupted supply of venous return from the patient. There are additional safety devices such as arterial filters (which are now optionally integrated within the oxygenator itself) and bubble detectors, but these will be discussed in more detail in Chapter 23. ECMO systems are “closed,” in that they do not have reservoirs; so every cubic centimeter of blood that is pumped into the patient must be replaced by a cubic centimeter coming into the venous return side. While many systems do have very small collapsible compartments used as either servo-regulators for roller pumps or compliance
chambers to minimize cavitation of air from the high negative pressures generated by centrifugal pumps, these are not reservoirs and do not have the capacity to store or supply blood volume. However, this also eliminates much of the stasis of blood within the system and is the reason ECMO can run on significantly lower levels of anticoagulation when compared to CPB.
chambers to minimize cavitation of air from the high negative pressures generated by centrifugal pumps, these are not reservoirs and do not have the capacity to store or supply blood volume. However, this also eliminates much of the stasis of blood within the system and is the reason ECMO can run on significantly lower levels of anticoagulation when compared to CPB.
Operation of the Oxygenator
The heart-lung machine is usually controlled by a perfusionist, who is responsible for maintaining adequate oxygen delivery to all the body’s tissues and organs during the period of support. Similarly, the ECMO circuit is maintained by personnel who perform similar roles on a longer-term basis. This involves all three highly interrelated components of support: the pump, the oxygenator, and temperature control. Providing adequate pump flow is the equivalent to the native cardiac output. Controlled hypothermia results in a protective effect during support or after acute ischemia/hypoperfusion by decreasing metabolic demands. And adequate gas exchange provides the needed oxygen to the tissues (provided adequate flow and adequate oxygen carrying capacity by hemoglobin) as well as carbon dioxide removal. The movement of O2 and CO2
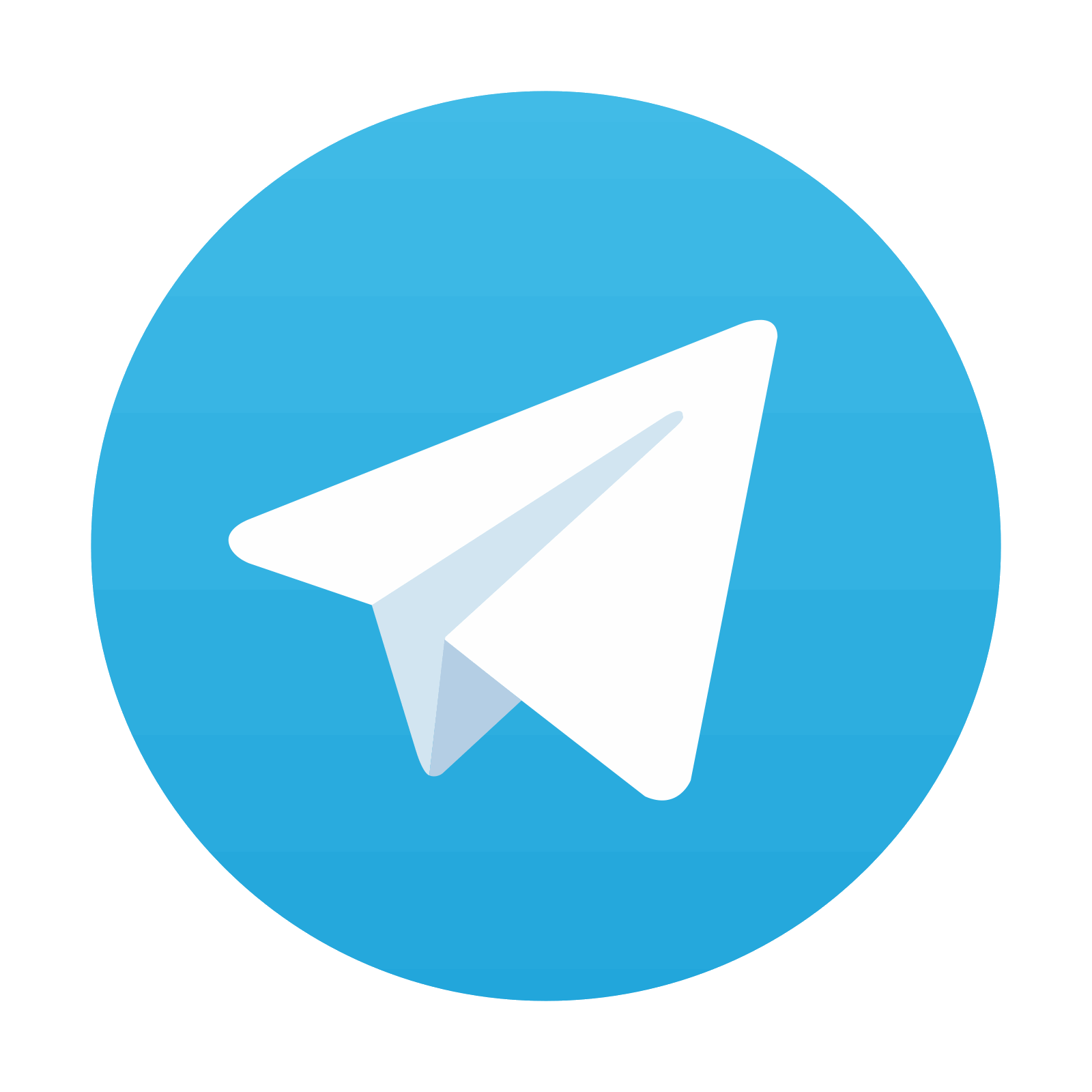
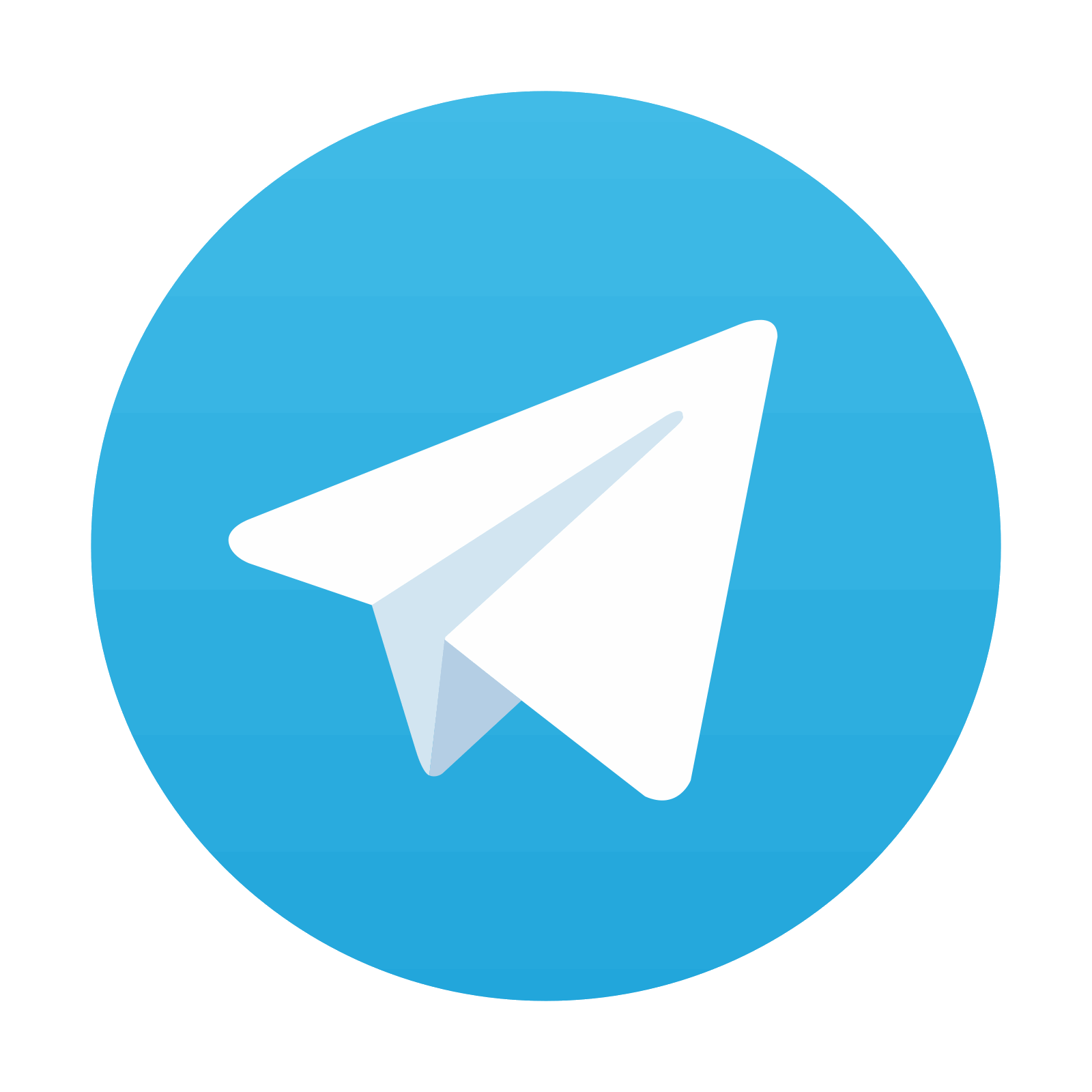
Stay updated, free articles. Join our Telegram channel
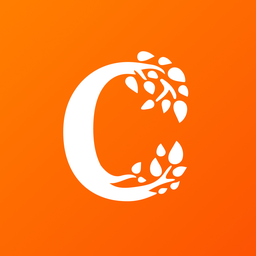
Full access? Get Clinical Tree
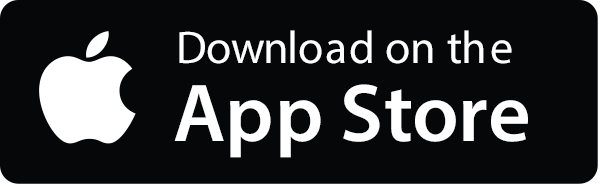
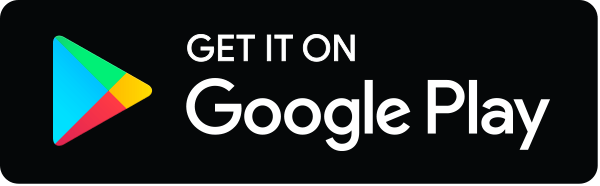
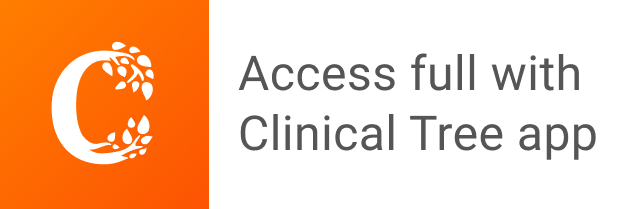