Abstract
Mechanical ventilation is central to critical care and came into routine practice after the polio outbreaks in the mid-20th century. A contemporary mechanical ventilator is a device made to deliver breaths using positive pressure (pressure above atmospheric level). The technology has become increasingly sophisticated in efforts to improve patient comfort and synchrony, as well to enhance ease of use. It is used for many indications, mainly for respiratory failure either from pulmonary or extrapulmonary sources, as well as to provide anesthesia. The most frequently used invasive modes in pediatric populations are synchronized pressure control ventilation with/without volume limitation and pressure support ventilation. While in special circumstances, such as congenital diaphragmatic hernia and severe hypoxemic respiratory failure, high frequency oscillatory ventilation may be a first choice, in most cases noninvasive ventilation and humidified high flow are increasingly used. However, positive pressure breathing can cause direct injury to lungs and has deleterious effects on hemodynamics. The overall goal therefore is to optimize patient needs and minimize risk of harm, while tailoring the ventilation strategy to the patient need. Hence, selection of right mode and strategy, optimization of respiratory mechanics and gas exchange, and provision of expert care are central to management of mechanical ventilation in critically ill children. These are addressed in this chapter.
Keywords
mechanical ventilation, noninvasive ventilation, high-frequency ventilation, neurally adjusted ventilation, gas humidification, complications of mechanical ventilation, hemodynamic effects of mechanical ventilation
History
Historical descriptions of artificial respiration include 18th-century devices for intubation, bag and mask ventilation, and application of negative extrathoracic pressure. The widespread use of mechanical ventilation (MV) did not occur until the polio outbreaks of the 1950s when iron lungs (negative pressure chambers) were deployed. Bjorg Ibsen, a Danish anesthesiologist, was the first to deploy positive pressure ventilation for the care of these patients as the iron lung did not prevent aspiration and was inefficient in the presence of significant atelectasis. Approximately 200 medical students manually ventilated patients via tracheostomies, and the overall mortality was 25%, compared with an anticipated rate of 90%. This laid the foundation for modern critical care medicine. Since then, devices for MV have become more sophisticated facilitating synchrony between the patient and the ventilator, the use of graphics, added measurements, and user friendliness.
Indications for Mechanical Ventilation
In most situations, the indications for MV are evident. The principal indications for MV in the critically ill are inadequate respiratory effort, or to recruit lung units and improve oxygenation. Causes of inadequate respiratory effort include a sustained increase in respiratory and metabolic demand (e.g., pneumonia, asthma, lung injury, sepsis, or metabolic acidosis), or underlying neuromuscular failure, such as acute or chronic paresis or fatigue.
MV is used during general anesthesia if respiration is inhibited or blocked by deep sedation, opioids, or neuromuscular blockade. Indications for intubation also include “protection” of the airway, when the patency of the airway is diminished from proximal airway obstruction due to edema or tumor, or is at risk from the aspiration of gastric contents or hemorrhage; in such situations, MV is usually added.
In addition to providing a “pumping” action that facilitates tidal gas exchange, MV is also used to provide elevated airway pressure, which recruits collapsed lung units (or maintains local recruitment), thereby improving oxygenation.
Finally, ventilation may be used in an emergency to provide hyperventilation when hypocapnia is temporarily sought in the management of acute intracranial hypertension.
Composition of a Mechanical Ventilator
The ventilator consists of a gas delivery system, in addition to several subsystems ( Fig. 34.1 ). The delivery system in turn consists of a breath controller, a mode controller, and a demand sensor. The breath controller generates the flow (i.e., the tidal volume [V T ]), which may be entirely derived from the ventilator, or in part, from the patient’s effort.

There are three elements at play in the delivery of a V T . First, the breath is triggered (by ventilator timing, or is initiated by the detection of patient inspiratory effort). Second, the flow of gas is controlled either by attaining a certain pressure, or volume, during inspiration. If “pressure” is targeted, then the inspiratory gas flow will vary depending on the difference between the pressure in the airway at the trachea (measured) and that in the alveoli (not measured), and will decrease as the inspiration progresses. If the “volume” is targeted, then the volume is delivered equally during each fraction of the inspiratory time (i.e., at a constant flow rate). Third, the ventilator “cycles off,” to end inspiration and permit (passive) exhalation; as with initiating inspiration, cycling off can be initiated by ventilator timing or by detecting the patient’s expiratory effort.
Where initiation or termination of inspiration is governed by the patient, effort sensors are key. These detect changes in pressure (or flow), and the sensitivity (how sensitive is the ventilator to change in airway pressure or flow) and responsiveness (speed of response by the ventilator to such a change) of “effort sensors” are major determinants of satisfactory patient-ventilator interaction.
Important additional components include gas blenders that mix air (21% O 2 ) and oxygen (100% O 2 ) to achieve the required F I O 2 . An “expiratory pressure generator” is required to maintain a certain pressure in the airway during expiration (termed: positive end-expiratory pressure, PEEP), to recruit atelectatic lung units, or to prevent their collapse.
Effective humidification and temperature control of the inspired gas is achieved by the use of special humidifiers, and the gas delivery circuit connects the ventilator to the interface (usually an endotracheal tube [ET], noninvasive ventilation mask, or ventilation hood).
Modes of Ventilation
Modes of MV can be described in terms of the type of “control” (pressure vs. volume) and the initiation of the breath ( Fig. 34.2 ).

The control reflects either volume or pressure. Volume control ventilation indicates that the V T is predetermined. Because the inspiratory time is fixed, the volume is delivered through inspiration at a constant flow (“square wave” pattern); thus, inspiratory pressure reflects any changes in lung mechanics. The V T is controlled at the set value by the ventilator, and is delivered independent of the chosen level of PEEP.
In pressure control ventilation the inspiratory pressure is constant; therefore, the pressure-time waveform is a “square wave” pattern. The flow (therefore the volume) of gas, which depends on the pressure difference between the ventilator (gas source) and the alveoli (gas destination), diminishes over time (as the lungs fill). In addition, any changes in compliance, resistance or patient effort will alter the flow (but not the pressure). The initiation and termination of a pressure control breath depend on timing, that is the rate (breaths per minute), and the duration of inspiration (T I ), which are set on the ventilator.
In pressure support ventilation, the profile of pressure and principles of flow are the same as in pressure control. However, the initiation of a breath needs to be triggered by an inspiratory effort by the patient; in contrast, termination of inspiration occurs when the inspiratory flow rate falls to 20%–30% (chosen by the clinician) of the peak (initial) inspiratory flow rate, which occurs when a patient attempts to exhale.
The “type” of breath is the second main factor, and this describes the interaction between the patient and the ventilator. Continuous mandatory ventilation (CMV) indicates that the ventilator provides all the work of breathing (WOB); indeed, there is no ability for the patient to breath as the ventilation is set by the clinician and cannot be altered by the patient.
Assist control (AC) indicates that the patient can initiate (trigger) the breath, but as soon as the inspiration has been initiated, the ventilator completes the breath. The clinician sets the “minimum” (or back-up) number of breaths per minute, which is the number of breaths that will occur if the patient makes no effort. However, each breath initiated by the patient will be completed by the ventilator (regardless of the rate). Weaning is difficult with this mode, because every breath results in a full V T —even if the AC rate is set to a low value—and this readily leads to hyperventilation.
With intermittent mandatory ventilation (IMV), the ventilator provides intermittent mandatory breaths based on the set minute ventilation; however, between these breaths, patients can make their own breaths that are not altered (assisted, completed, or inhibited) by the ventilator. In contrast to AC, patient breaths are not assisted by the ventilator; therefore, weaning the IMV rate means that the patient can make intermittent breaths of variable size without resultant hyperventilation.
Modern IMV is “synchronized” so that the ventilator sets windows of time each minute during which if a sufficient V T is not completed by the patient it will be completed by the ventilator; synchronization means that these windows are reset every time an adequate V T has occurred. This helps achieve better patient–ventilator synchrony.
Continuous spontaneous ventilation refers to a simple mode wherein most of the WOB is done by the patient; the ventilator provides constant pressurization of the circuit (continuous positive airway pressure, CPAP), on which pressure support (see above) can be superimposed.
Although important for understanding the classification of ventilators, pure forms of CMV or IMV are almost never used in contemporary practice.
Noninvasive Ventilation
Noninvasive ventilation (NIV) describes the delivery of mechanical support through an interface (e.g., nasal prongs or mask, face mask, or helmet) and without the need for an ET. NIV offers the ability to reduce patient WOB and improve respiratory gas exchange while avoiding the risks and complications related to the placement of an ET, including sedation and neuromuscular blockade. In addition, it can easily be initiated during transport or in settings where intubation in not commonly performed.
Conventional NIV is delivered using nasal cannula, nasal mask, full face mask, and helmet. A full-face mask or helmet more reliably provides positive airway pressure, but it may cause agitation, especially in infants and younger children, and has a greater dead-space (requiring high flows to clear the CO 2 ). A nasal cannula or mask is often better tolerated but a pressure leak due to a poor interface seal or through the mouth may limit effectiveness.
NIV is designed to either deliver CPAP: Continuous distending pressure for the whole respiratory system) or bilevel positive airway pressure (BiPAP: two levels of positive airway pressure—inspiratory and expiratory). BiPAP is either synchronized (spontaneous) with each patient effort or with a defined back up rate (Timed).
NIV is particularly used in bronchiolitis, asthma, pneumonia, pulmonary edema, cystic fibrosis, acute chest syndrome, laryngotracheomalacia, and acute hypoxemic failure. Contraindications to the use of NIV include cardiopulmonary arrest, the inability to protect upper airway, poor neurological status, shock (requiring escalating vasopressors), upper gastrointestinal bleed, facial injuries, and untreated pneumothorax. The appropriate selection of interface and mode of NIV are key to optimizing support for children. It is clinically titrated to WOB, heart rate, subjective comfort, and oxygenation. Frequently BiPAP is used for ventilation failure; therefore, the effect is measured as efficacy of CO 2 removal.
Air leak syndrome, aspiration, and hemodynamic instability from cardiac preload reduction are serious but rare complications, whereas gastric distension and facial skin breakdown are more common. The inability to humidify the gas may lead to nasal/oral mucosal damage. Eye irritation, trauma, and inflammation can also result from improper mask fit and continuous exposure to high gas flow.
Heated high flow O 2 is a newer delivery system used to deliver gas flow rates of up to 8 L/min in an infant (or about 60 L/min in large child). It allows delivery of flow rates higher than the patient’s peak inspiratory flow with fixed FiO 2 . It generates PEEP of 2–5 cm H 2 O, reduces WOB, improves tolerance of the oxygen delivery system, and reduces the need for intubation. It is particularly helpful in children with bronchiolitis and airspace disease without ventilation failure. Importantly, it does not guarantee a fixed airway pressure, and thus may generate lower transpulmonary pressure than NIV, when a patient adds a significant inspiratory effort to the external ventilator support.
High-Frequency Ventilation
High-frequency ventilation (HFV) applies continuous distending pressure to maintain lung expansion, and superimposes small V T s at a rapid rate; the main type of HFV used is high-frequency oscillatory ventilation (HFOV). The small V T s result from oscillating air movements produced by the ventilator diaphragm or piston at frequencies of 600–900 breaths per minute (10–15 Hz), which result in both positive (inspiratory) and negative (expiratory) pressure fluctuations.
Two major concepts differentiate HFOV and so-called conventional ventilation (although HFOV has been in use for over 40 years). First, CO 2 is cleared despite using a V T that is less than physiological dead-space, a phenomenon that is not possible using standard “bulk flow” kinetics. However, with HFOV, multiple mechanisms contribute to CO 2 removal. Second, CO 2 removal (which is increased by greater V T , but not in a linear relationship), is increased by decreasing the frequency; this counterintuitive finding is because with a lower rate, the time to develop a V T is increased, and this increases CO 2 clearance.
In most circumstances, the major use for HFOV is lung recruitment, and this is primarily a function of the mean airway pressure employed, and is assessed in terms of oxygenation response. The other key adjustments are the amplitude and frequency of the pressure wave, to achieve CO 2 clearance, as well as the inspired O 2 concentration (FiO 2 ).
HFOV has a long history in pediatric critical care. One subset of potential priority is ventilated children with neonatal pulmonary hypertension (e.g., congenital diaphragmatic hernia), where the major rationale is enhanced CO 2 clearance (below).
Neurally Adjusted Ventilator Assistance
Conventional MV tracks changes in airway pressure (and flow), and the assumption is that these parameters account for changes in the patient’s respiratory “need” (i.e., respiratory drive). However, increased respiratory drive may not translate into increased diaphragmatic contraction, perhaps due to partial paralysis or muscle weakness. Further, increased diaphragm contractility may not translate into an adequately negative deflection of the pressure–time curve during expiration, due to lung injury and poor pressure transmission from the pleural space to the airway. Because the conventional ventilator only senses changes in airway pressure (or flow), there may be a significant discrepancy between the patient “need” (i.e., phrenic nerve output to the diaphragm) and the level of support supplied by the ventilator.
Neurally adjusted ventilator assistance (NAVA) senses the electric activity of the diaphragm (EA di ), which is independent of ventilator, circuit, and respiratory mechanics, using a catheter embedded in a gastric tube. When electrical activity is greater than a set threshold (0.5 µV) a mechanical breath is delivered; the size of the breath (of level of pressure) is based on the amplitude of EA di and the level of assistance determined by the clinician. This results in breath-to-breath variation of V T , and adjustment is made to the assist level such that a satisfactory V T is delivered and a diminished EA di signal is detected that reflects lessened patient demand. This approach should improve patient comfort and prevent over or under ventilation, relative to neutrally determined need.
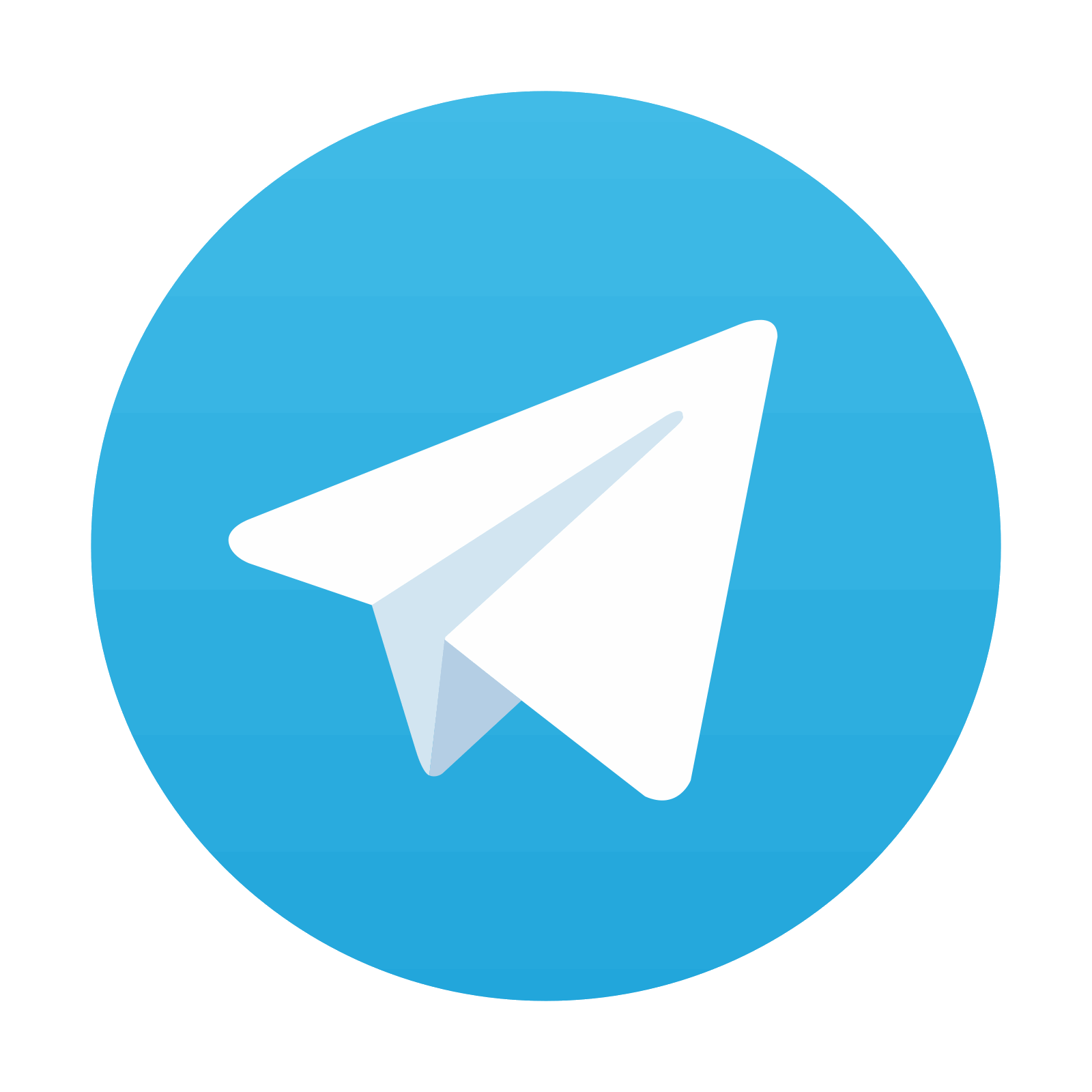
Stay updated, free articles. Join our Telegram channel
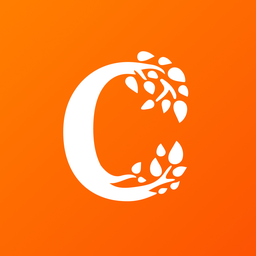
Full access? Get Clinical Tree
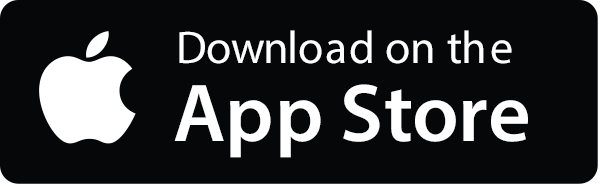
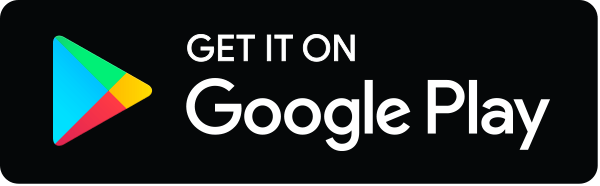